12 Chapter 12: Evolution
Chapter Outline
12.1 Evidence for Evolution
- Fossil Record
- Homology
- Biogeography
12.2 Mechanism of Evolution: Natural Selection
- Principles of Natural Selection
- Demonstrations of Natural Selection
12.3 Other Mechanisms of Evolution
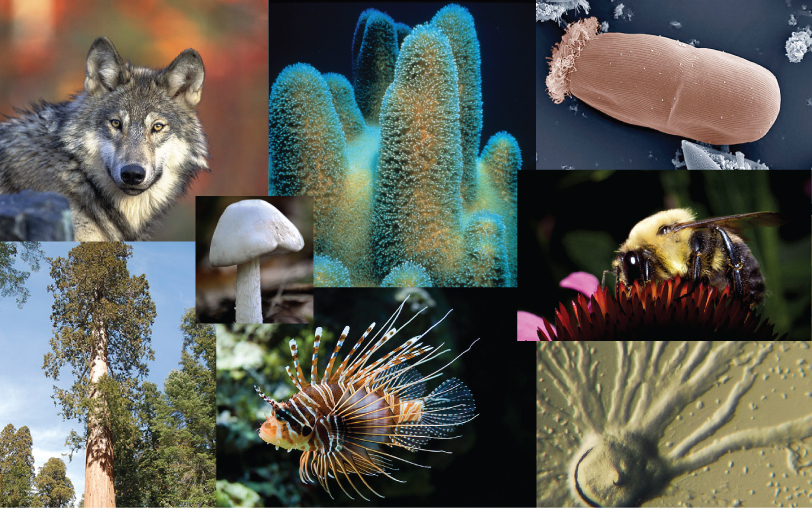
All species of living organisms—from the bacteria on our skin, to the trees in our yards, to the birds outside—evolved at some point from a different species. Although it may seem that living things today stay much the same from generation to generation, that is not the case: evolution is ongoing. Evolution is change over time—it is the process through which the characteristics of species change and through which new species arise.
The theory of evolution is the unifying theory of biology, meaning it is the framework within which biologists ask questions about the living world. Its power is that it provides direction for predictions about living things that are borne out in experiment after experiment. The Ukrainian-born American geneticist Theodosius Dobzhansky famously wrote that “nothing makes sense in biology except in the light of evolution”[1] He meant that the principle that all life has evolved and diversified from a common ancestor is the foundation from which we understand all other questions in biology. This chapter will explain some of the mechanisms for evolutionary change and the kinds of questions that biologists can and have answered using evolutionary theory.
Learning Objectives
- You will be able to describe the various lines of evidence for the evolution of life, including the fossil record, biogeography, vestigial traits, and various types of homologies.
- You will demonstrate the process of evolution, which results in increasing the diversity and complexity of life. Specifically, you will understand and provide examples of adaptation through natural selection, which requires the following factors:
- operates on populations in specific environmental contexts
- necessary because of the competition for limited resources
- relies on biological processes that produce of new variation
- transfers new variation through the mechanisms of inheritance
- results in differential fitness (differences in survival and reproduction)
12.1 Evidence for Evolution
The evidence for evolution is compelling and extensive. Looking at every level of organization in living systems, biologists see the signature of past and present evolution. Darwin dedicated a large portion of his book, On the Origin of Species, identifying patterns in nature that were consistent with evolution and since Darwin our understanding has become clearer and broader.
12.1.1 Fossil Record
Fossils are the preserved partial remains (usually only hard bony tissue or shells survive but occasionally soft tissue remains are also preserved) of living organisms that have been buried and protected from the elements that cause decomposition and degradation. The remains are slowly transformed as minerals from the surrounding sediments slowly replace the proteins in the tissues. Because bony tissue and shells is already partially mineralized (in the case of bones, the most common mineral is calcium) while the organism is still alive, they preserve very well.
Fossils provide solid evidence that organisms from the past are not the same as those found today; these fossils show a progression of evolution. Scientists determine the age of fossils and categorize them all over the world to determine when the organisms lived relative to each other. The resulting fossil record tells the story of the past, and shows the evolution of form over millions of years (Figure 12.2). For example, highly detailed fossil records have been recovered for sequences of species showing the evolution leading up to modern horses. The fossil record of horses in North America is especially rich and many contain transition fossils – fossils that show intermediate anatomy between earlier and later forms. The fossils show that horse-like organisms first evolved from a dog-sized ancestor about 55 million years ago. This ancestor gave rise to the first horse-like species, which slowly evolved into successively larger species. This series of fossils also shows a change in the size and shape of their teeth to be better-suited for grazing on tough, dry grass instead of soft leaves. Also, the horse lineage shows a change towards long, strong legs that are allow the horses to run quickly for long periods of time on hard-packed, dry ground to escape predators. These changes in the anatomy of their teeth and legs are adaptations resulting from a gradual drying trend that changed the landscape from a forested one to a prairie. An adaptation is something that “matches” an organism to the environment. In a prairie environment, grass is the prevalent plant food resource so the horse must have large teeth capable of grazing on grass. They must have long strong legs because they must run away from rather than hide from predators because they are highly visible in an environments with few trees.
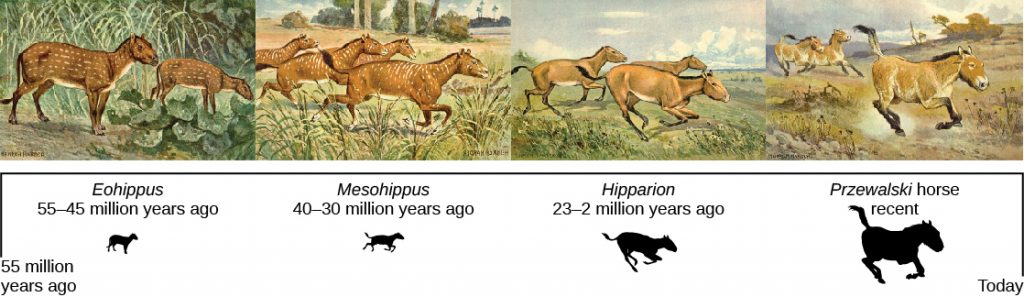
12.1.2 Homology
Another type of evidence for evolution is the presence of structures in organisms that share the same basic form, which are referred to as homologies. The word homology means a set of similar structures, which can be large such as similar bones or microscopic such as similar proteins. The similarities in structures among a group of species indicate that they had a common ancestor. Each species may have slight differences in structure because they followed different evolutionary pathways, but the basic body plan that they each inherited from a common ancestor is evident.
Anatomical Homology
An anatomical homology is a structure or set of structures, such as limb bones, that are very similar in form and position across several species. For example, the bones in the appendages of a human, dog, bird, and whale all share the same overall construction (Figure 12.3). That similarity is the results of inheriting the basic plan from a common ancestor. Over time, evolution led to some alterations in the shapes and sizes of these bones in different species, but they have maintained the same overall layout.
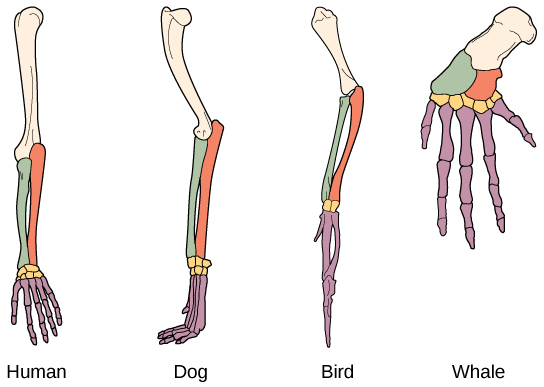
The broad, general function of some of these forelimbs is the same (locomotion), although the precise function is slightly different for each species: dog forelimb = walking/running, bird forelimb = flying, whale forelimb = swimming. The function of the human forelimb is very different – it gives us the ability to carry and manipulate objects. Yet, the anatomy (shape and position) of the forelimbs of these four different species is broadly similar and indicates that they all inherited the same basic plan from a common ancestor millions of years ago.
Some structures serve an important function in one or more species but have no apparent function in another species and appear to be residual parts from a past ancestor. For example, some snakes have pelvic bones despite having no legs because they descended from reptiles that did have legs. These unused structures without function are called vestigial traits. Other examples of vestigial structures are wings on flightless birds (which may have other functions), leaves on some cacti, traces of pelvic bones in whales (Figure 12.4), and the sightless eyes of cave animals.
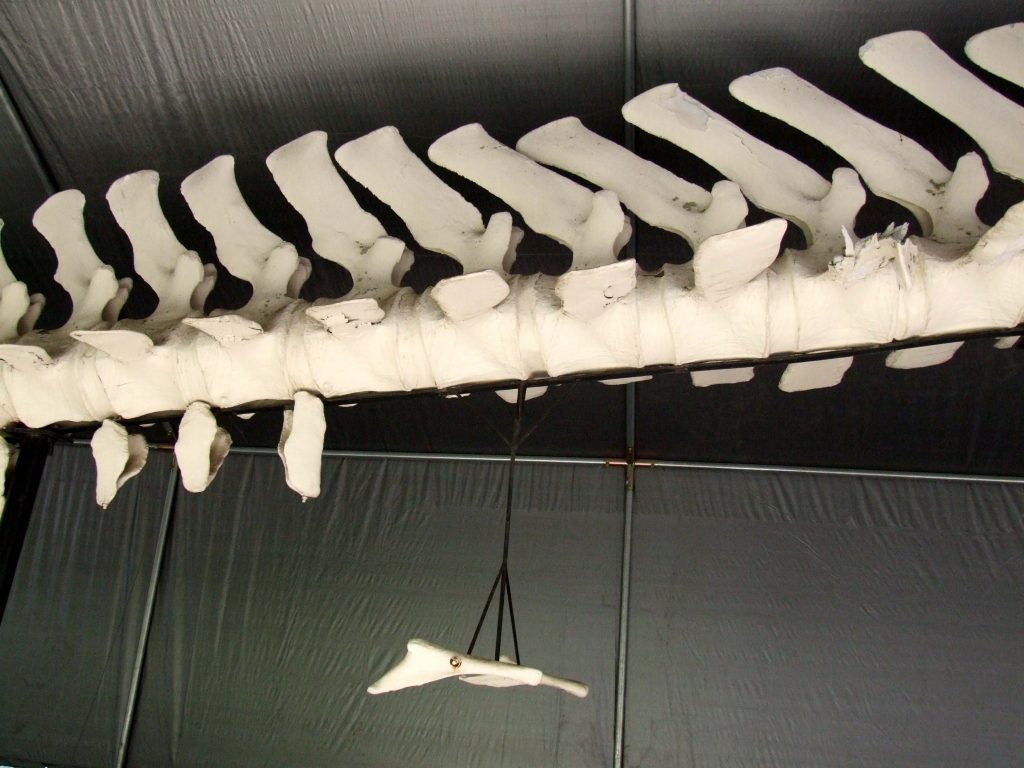
Another line evidence of evolution is the convergence (coming together) of form in organisms that share similar environments. These similarities are not due to inheritance from a common ancestor. These structures perform similar functions but they have some essential differences. For example, species of unrelated animals, such as the arctic fox and ptarmigan (a bird), living in the arctic region have temporary white coverings during winter to blend with the snow and ice (Figure 12.5). The similarity occurs not because of common ancestry, indeed one species inherited fur from its ancestor and the other inherited feathers. Instead, similar evolutionary pressures – the benefits of not being seen by predators – resulted in the superficial similarity of a white covering.
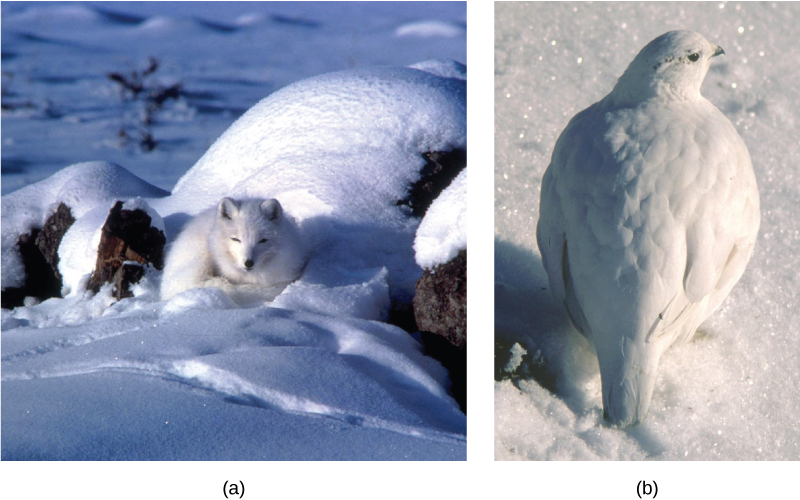
Developmental Homology
Developmental homology is a similarity in the patterns of growth and development between species. In particular, embryology, the study of the prenatal development of the anatomy of organism, provides strong evidence for the relatedness between now widely divergent groups of organisms. The embryos of vertebrates (organisms with a bony spinal column) begin a similar basic body plan and then each species develops specialized anatomy as gestation progresses (Figure 12.6). The reason early embryos of distantly related species are often so similar is that small changes during embryonic development can result in greatly amplified differences by the time development is complete.
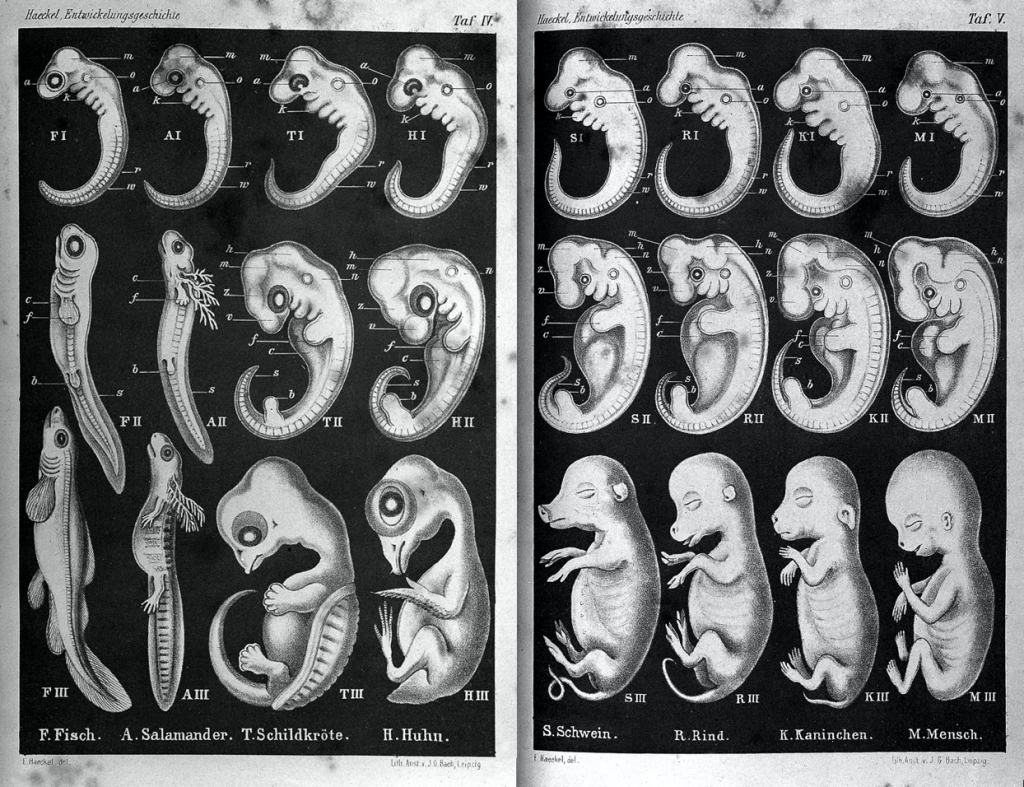
In addition to developing specialized traits, some species lose some of the traits of the basic vertebrate body plan of the common ancestor. These traits appear in the early embryonic stages and then disappear by the time the organism is fully developed. For example, all vertebrate embryos, including humans, exhibit gill slits at some point in their early development. These disappear in the adults of terrestrial (land-dwelling) groups, but are maintained in adult forms of aquatic groups such as fish and some amphibians. Great ape embryos, including humans, have a tail structure during their embryonic development that is lost by birth.
Molecular Homology
Like anatomical structures, the structures of the molecules of life reflect evolutionary processes over time. Molecular homologies are similarities in DNA sequences and consequently, the structure of proteins. Evidence of a common ancestor for all of life is reflected in the universality of DNA as the source of biological information and the near universality of the genetic code (i.e. across all forms of life, each codon consistently represents a particular amino acid or other information such as “stop transcribing”). In addition, the machinery and processes of DNA replication and gene expression is universal across life on earth. The first fundamental divisions in life between the three domains are reflected in major structural differences in their cells, yet the differences are rather conservative among key structures such as the structures of membranes, the structure of DNA/RNA molecules, the components of ribosomes, and the process of protein synthesis. Thus, there is a “basic body plan” of sorts with regard to the flow of biological information. In addition, there are some proteins, and consequently DNA sequences that code for these proteins, that are the same or very similar across a wide range of species. For instance, a sodium channel, a protein on a cell’s membrane that regulates the amount of sodium entering and exiting the cell, can be very similar in structure and function in very distantly related species.
Because the DNA sequence of a species evolves slowly over time, the relatedness of groups of organisms is reflected in the similarity of their DNA sequences—exactly the pattern that would be expected from descent and diversification from a common ancestor. Among the great apes, which includes humans, the genetic differences are remarkably small (Figure 19.7). A comparison of the DNA sequences shows that we share a most recent common ancestor with chimpanzees, followed by the next most recent common ancestor with gorillas. The DNA sequences also demonstrate that a small change in a single gene can have a large effect on the structure and function of a given protein, and thus the functioning of the entire organism.
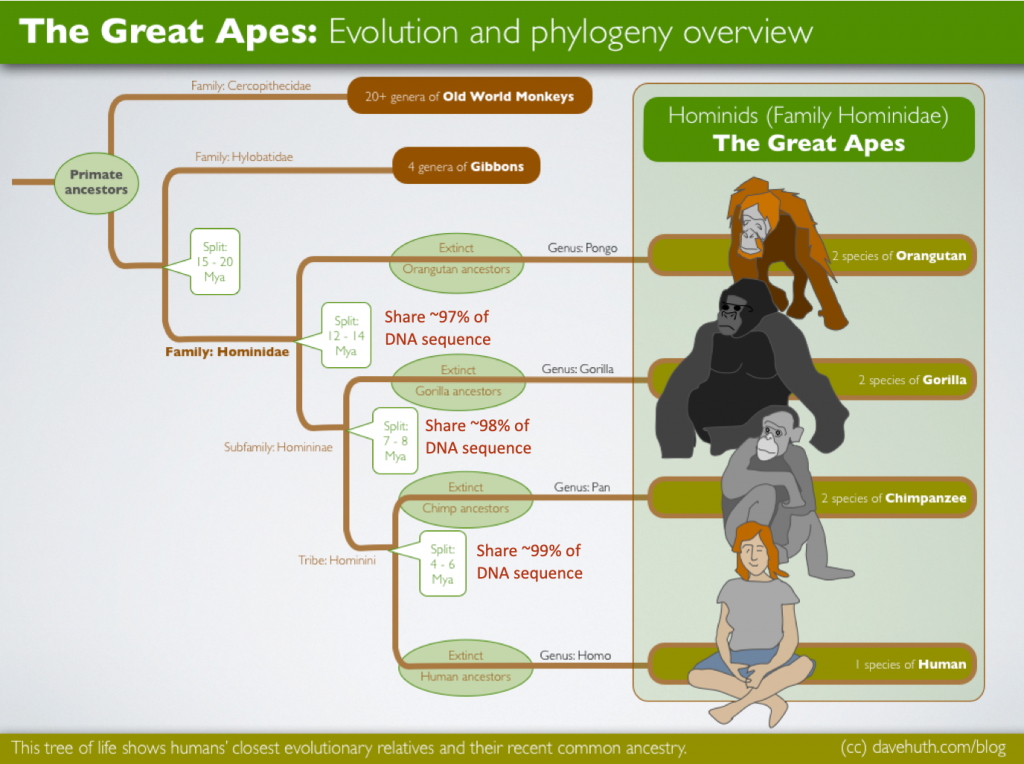
12.1.3 Biogeography
Biogeography is the study of the geographic distribution of organisms on the planet. This distribution shows patterns that are best explained by evolution in conjunction continental drift – the movement of the tectonic plates over geological time. Broad groups that evolved before the breakup of the supercontinent Pangaea (about 200 million years ago) are distributed worldwide. Groups that evolved since the breakup appear uniquely in regions of the planet, for example the unique flora and fauna of northern continents that formed from the supercontinent Laurasia and of the southern continents that formed from the supercontinent Gondwana. The presence of Proteaceae in Australia, southern Africa, and South America is best explained by the plant family’s presence there prior to the southern supercontinent Gondwana breaking up (Figure 12.8).
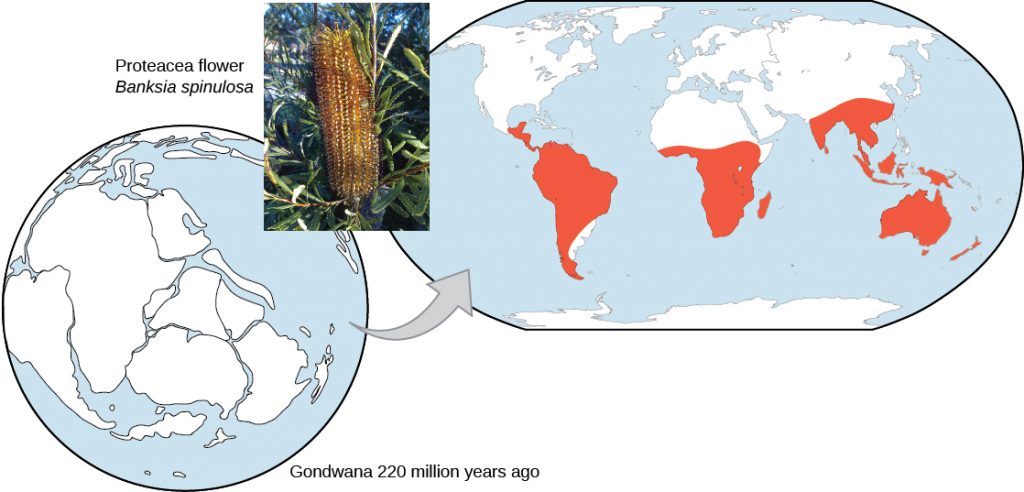
12.2 Mechanisms of Evolution: Natural Selection
Thus far, we have reviewed the evidence for evolution – the fossil record, three types of homologies, and biogeography. This evidence demonstrates that species change over time. However, we have not examined how species change over time or the mechanisms of evolution. We will devote most of our time to the most prevalent evolutionary force – natural selection.
12.2.1 Principles of Natural Selection
The actual mechanism for evolution was independently conceived of and described by two naturalists, Charles Darwin and Alfred Russell Wallace, in the mid-nineteenth century. Importantly, each spent time exploring the natural world on expeditions to the tropics. From 1831 to 1836, Darwin traveled around the world on H.M.S. Beagle, visiting South America, Australia, and the southern tip of Africa. Wallace traveled to Brazil to collect insects in the Amazon rainforest from 1848 to 1852 and to the Malay Archipelago from 1854 to 1862. Darwin’s journey, like Wallace’s later journeys in the Malay Archipelago, included stops at several island chains, the last being the Galápagos Islands west of Ecuador (Figure 12.9).
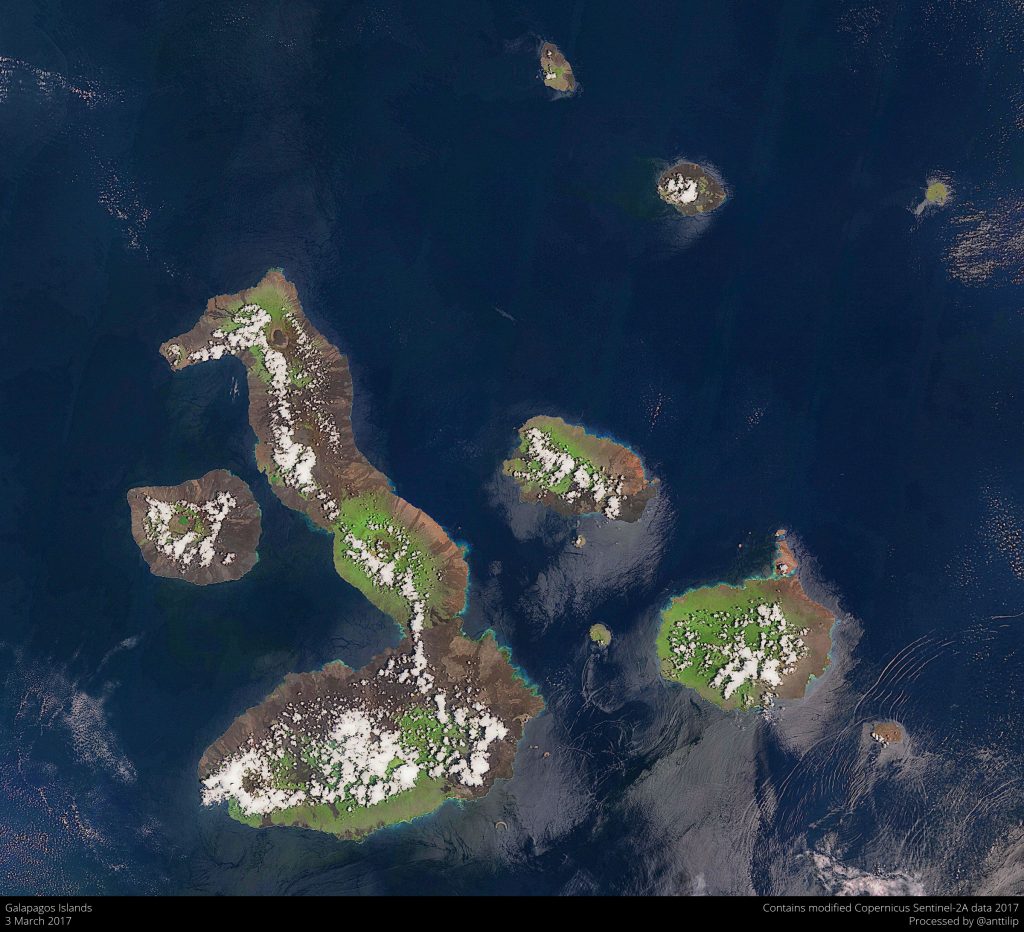
On these islands, Darwin observed species of organisms on different islands that were clearly similar, yet had distinct differences. For example, the ground finches inhabiting the Galápagos Islands comprised several species that each had a unique beak shape (Figure 12.10). He observed both that these finches closely resembled another finch species on the mainland of South America and that the group of species in the Galápagos formed a graded series of beak sizes and shapes, with very small differences between the most similar. Furthermore, the differences in beak size and shape reflected differences in function – e.g. the larger beaks were better suited for breaking hard seed cases whereas smaller beaks were better for obtaining small, delicate seeds. These various beak sizes are adaptations – traits that were best-suited for the organism’s environmental conditions. Darwin imagined that the island species might be all species modified from one original mainland species. In 1860, he wrote, “Seeing this gradation and diversity of structure in one small, intimately related group of birds, one might really fancy that from an original paucity of birds in this archipelago, one species had been taken and modified for different ends.”[2]
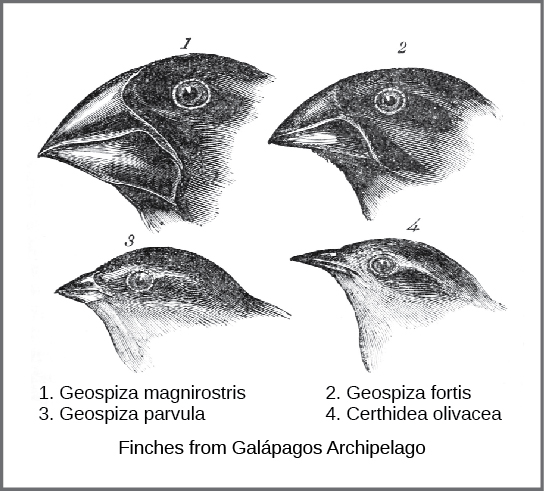
Wallace and Darwin both observed similar patterns in other organisms and independently conceived a mechanism to explain how and why such changes could take place.
Darwin and Wallace reasoned that offspring with inherited traits that allow them to best compete for limited resources will survive and have more offspring than those individuals with variations that are less able to compete. Because characteristics are inherited, these traits will be better represented in the next generation. This will lead to change in populations over generations in a process that Darwin called “descent with modification.”
Darwin called this mechanism natural selection – natural forces cause changes in a species such that it becomes better adapted to its environment. Natural selection is the inevitable outcome of five factors that operated in nature:
- Variation
- Heritability
- Environmental context
- Competition
- Differential reproductive success
We’ll explore each of these factors in greater depth.
Variation
Natural selection can only take place if there is variation, or differences, among individuals in a population. Within every population of a species, there is variation in their traits. All the individuals in a population might look very similar, but not exactly the same. We can see this variation in any population, including humans.
Genetic diversity in a population comes from two main sources: mutation and sexual reproduction. Mutation, a change in DNA, is the ultimate source of new alleles or new genetic variation in any population. An individual that has a mutated gene might have a different trait than other individuals in the population. However, this is not always the case. A mutation can have one of three outcomes on the organisms’ phenotype (anatomy, physiology, and/or behavior):
- A mutation may affect the phenotype of the organism in a way that gives it reduced fitness—lower likelihood of survival, resulting in fewer offspring.
- A mutation may produce a phenotype with a beneficial effect on fitness.
- Many mutations, called neutral mutations, will have no effect on fitness
Heritability
Many of the traits of organisms are inherited, or passed from parent to offspring. Importantly, these differences must have some genetic basis; otherwise, selection will not lead to change in the next generation. If a trait is acquired during an organism’s lifetime and is not coded in its DNA, the offspring will not receive this information and it will not develop this trait. For instance, if someone loses a finger in an accident, their children will not be born being a finger. If an individual has a large amount of muscle mass due to high-quality nutrition and exercise, their children will not inherit a large amount of muscle mass (note that social and physical environments are often inherited among humans, so parents who eat wells and exercise frequently will likely pass along this lifestyle to their children; however, this sort of cultural inheritance is not biological evolution).
Only heritable traits, or traits that are coded in the organisms’ DNA, can evolve in a population from generation to generation. For instance, if a parent’s genotype is homozygous for a large beak (i.e. two alleles for a large beak on homologous genetic loci), its offspring will inherit an allele for a large beak (whether the offspring ends up with a large beak as part of its phenotype depends on which allele it inherits from the other parent – review the section on inheritance in Chapter 8 for more details).
Environmental Context
There is a wide range of environmental conditions across the planet. Each environment present unique challenges to the species inhabiting it. For instance, some environments are very cold while other environments are very dry. The environmental context is crucial to natural selection. Some of the variation in a population is advantageous is one particular environmental context while it would be disadvantageous in another environmental context. Species who inhabit a desert environment need to be able to retain whatever small amount of water that is available (Figure 12.11).
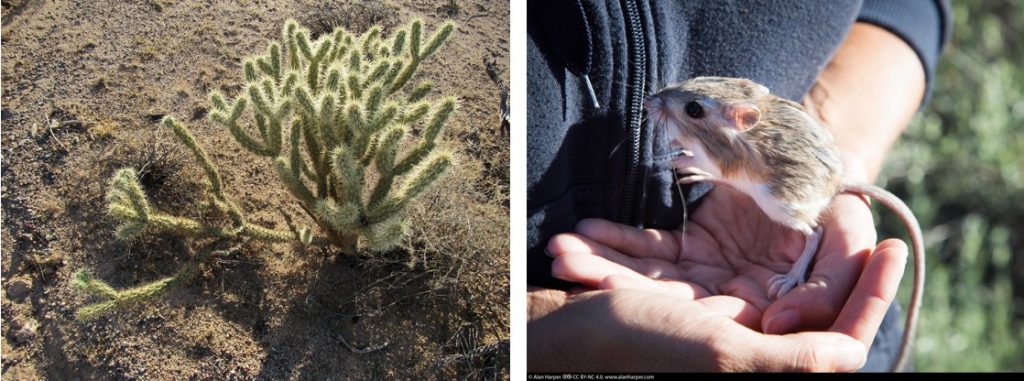
A different environmental context would result in different adaptations. A tropical rainforest has plenty of water but there are other challenges: trees will grow very tall or have very large leaves to obtain sufficient sunlight while many of the animals have adaptations to climbing large trees (Figure 12.12).
Figure 12.12 Tropical rainforest adaptation
Competition
Darwin and Wallace both observed that organisms reproduce more offspring than are able to survive. There is always competition: the resources available in the environment for survival and reproduction are limited and the capacity for reproduction in all organisms outstrips the availability of resources to support their numbers.
Competition can occur within a species, such as the trees in a tropical rainforest competing for sunlight and soil nutrients (Figure 12.12). Competition can also occur between species if they rely on the same resource, such as between African lions and spotted hyenas (Figure 12.13).
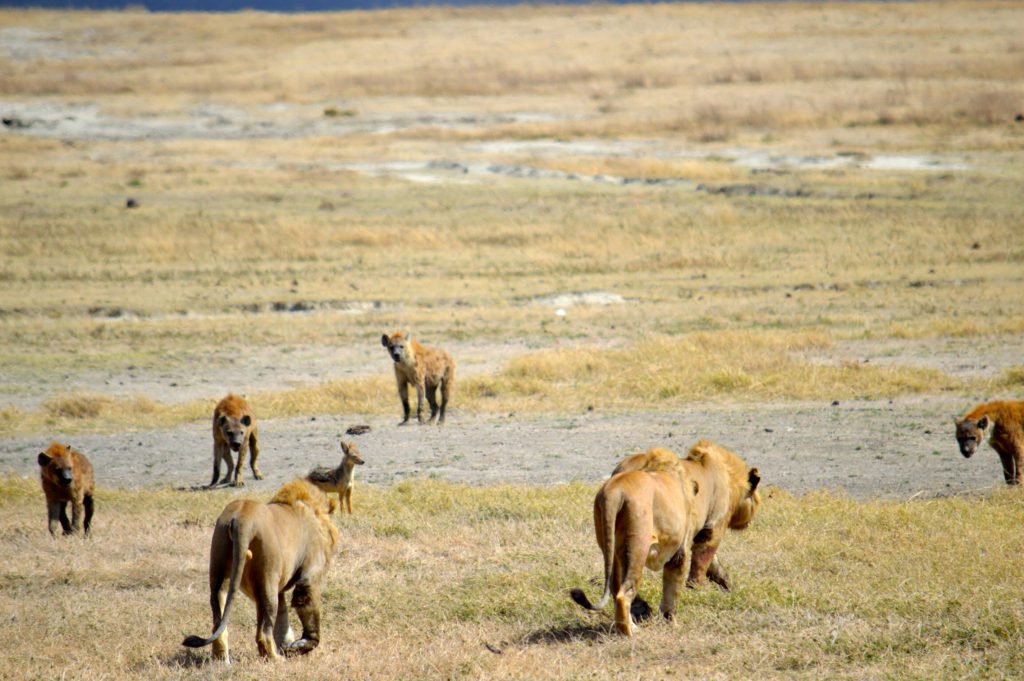
Differential Reproductive Success
In any given population, some individuals survive longer and/or have more offspring than other individuals in the population. From an evolutionary standpoint, survival alone is not enough for natural selection. The number of surviving offspring an organism has determines whether and how prevalent its genetic material is in next generation. Differential reproductive success simply means that individuals within a population have different numbers of offspring (Figure 12.14). Darwin referred to reproductive success as fitness: how many surviving offspring an organism has. The greater the number of offspring, the higher the fitness. Thus, in evolutionary biology, fitness does not refer to strength or physical conditioning as it commonly means in other contexts. Implicit to fitness is the need for survival – organisms who are more fit must have had a better chance of survival because dead organisms do not reproduce.
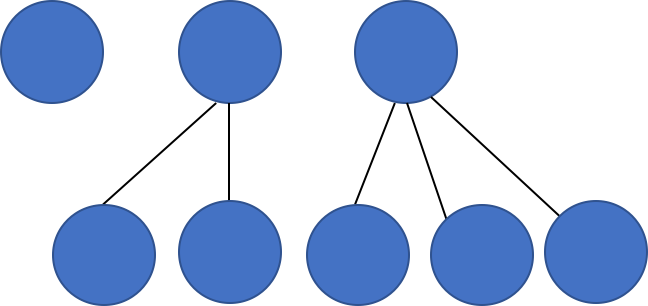
Summary of Natural Selection
At the mid-nineteenth century, Darwin and Wallace were not aware of genetics so they did not know the source of all new genetic variation as we do now (mutation), but they did observe that variation exists in every natural population. They did not know the precise mechanism of inheritance (reviewed in Chapter 8), but they did observed that offspring inherited traits from their parents. From these observations, Darwin and Wallace reasoned that offspring with inherited characteristics that allow them to best compete for limited resources will survive and have more offspring than those individuals with variations that are less able to compete. They further noted that the environmental context is key for determining which traits are selected for and which are selected against (Figure 12.15).
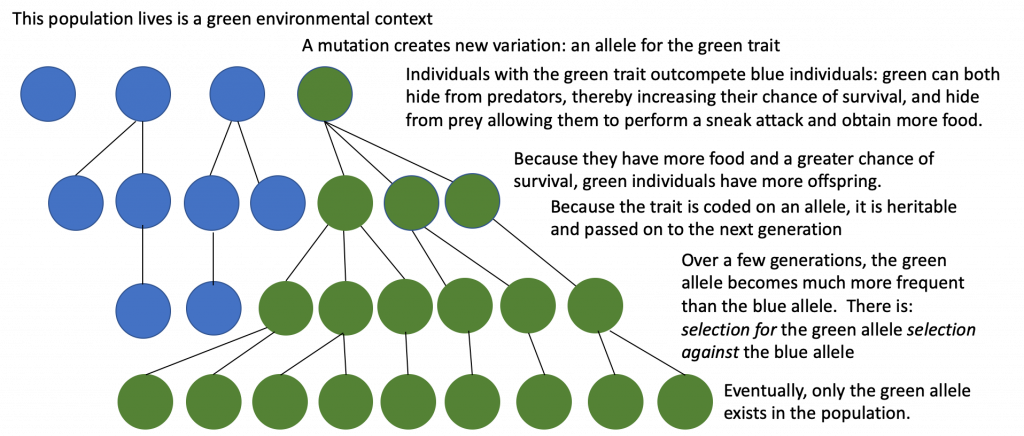
Evolution is sometimes defined as changes in allele frequencies from generation to generation. Allele frequencies refer to the percentage of each allele in a population. For example, in a hypothetical population, 65% of the alleles are blue and 35% are green. A change in allele frequencies means that these percentages have changed from one generation to the next. So, in the following generation, the hypothetical population could have 90% blue alleles and 10% green alleles.
There are several ways the allele frequencies of a population can change. One of those ways is natural selection. If a given allele confers a phenotype that allows an individual to have more offspring that survive and reproduce, that allele, by virtue of being inherited by those offspring, will be in greater frequency in the next generation. Since allele frequencies always add up to 100 percent, an increase in the frequency of one allele always means a corresponding decrease in one or more of the other alleles. Highly beneficial alleles may, over a very few generations, become “fixed” in this way, meaning that every individual of the population will carry the allele. Similarly, detrimental alleles may be swiftly eliminated from the gene pool, the sum of all the alleles in a population. Population geneticists track how selective forces change the allele frequencies in a population over time, which can give scientists clues regarding the selective forces that may be operating on a given population.
12.2.2 Demonstrations of Natural Selection
Galápagos Finches
Demonstrations of evolution by natural selection can be time consuming. One of the best demonstrations has been in the very birds that helped to inspire the theory, the Galápagos finches. Peter and Rosemary Grant and their colleagues have studied Galápagos finch populations every year since 1976 and have provided important demonstrations of the operation of natural selection. The Grants found changes from one generation to the next in the beak shapes of the medium ground finches on the Galápagos island of Daphne Major. The medium ground finch feeds on seeds. The birds have inherited variation in the bill shape with some individuals having wide, deep bills and others having thinner bills. Large-billed birds feed more efficiently on large, hard seeds, whereas smaller billed birds feed more efficiently on small, soft seeds. During 1977, a drought period altered vegetation on the island. After this period, the number of seeds declined dramatically: the decline in small, soft seeds was greater than the decline in large, hard seeds. The large-billed birds were able to survive better than the small-billed birds the following year. The year following the drought when the Grants measured beak sizes in the much-reduced population, they found that the average bill size was larger (Figure 12.16). This was clear evidence for natural selection (differences in survival) of bill size caused by the availability of seeds. The Grants had studied the inheritance of bill sizes and knew that the surviving large-billed birds would tend to produce offspring with larger bills, so the selection would lead to evolution of bill size. Subsequent studies by the Grants have demonstrated selection on and evolution of bill size in this species in response to changing conditions on the island. The evolution has occurred both to larger bills, as in this case, and to smaller bills when large seeds became rare.
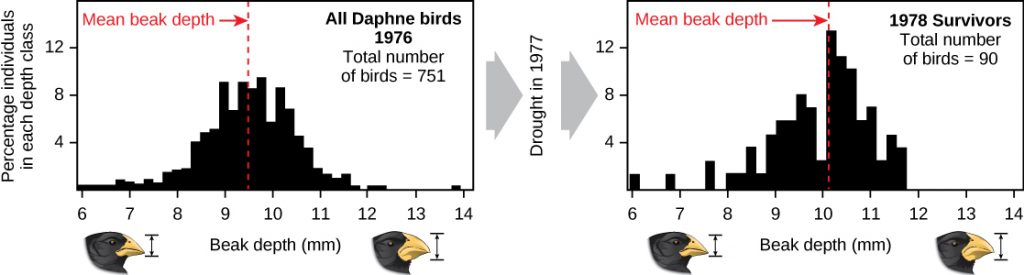
Peppered Moths
Another demonstration of natural selection occurred in the United Kingdom with the peppered moth. After the Industrial Revolution, some areas of the country such as Manchester experienced heavy pollution due to the burning of coal for fuel. The studies of changes in wing coloration in the peppered moth from mottled white to dark in response to soot-covered tree trunks and then back to mottled white when factories stopped producing so much soot is a classic example of studying evolution in natural populations (Figure 12.17).
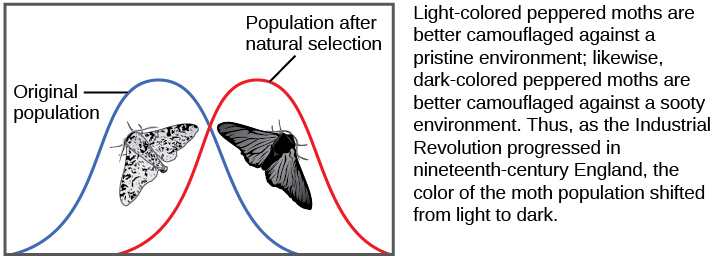
Sickle-Cell Anemia
Sickle-cell anemia is a classic example of natural selection operating in a human population. Sickle-cell anemia is a disease caused by a mutation of a single base-pair of the gene for hemoglobin, the protein inside of red blood cells that carries oxygen. The unique sequence of base pairs in a strand of DNA encodes (carries) the information for the sequence of amino acids that make up a given protein. Any change in the DNA sequence may lead to a change in one or more of the amino acids along chain, further leading to a change in the protein’s structure and function. The hemoglobin protein consists of a chain of 147 amino acids. In sickle cell anemia, there is a point mutation (single base-pair change) in the hemoglobin gene: adenine is changed to thymine, which results in the substitution in a single amino acid (from glutamic acid to valine) along the chain. This single amino acid chain results in a non-functional hemoglobin protein.
Red blood cells are normally biconcave, or disc-shaped. In the case of sickle-cell anemia, the hemoglobin proteins are misshapen, further causing the red blood cells distort into a crescent or “sickle” shape (Figure 12.18a). Sickled red blood cells are not able to carry sufficient oxygen. This can lead to a myriad of serious health problems, such as breathlessness, dizziness, headaches, and abdominal pain for those who have this disease. Furthermore, these abnormally shaped cells can become lodged in narrow blood vessels because they are unable to fold in on themselves to squeeze through, blocking blood flow to tissues and causing a variety of serious problems from painful joints to delayed growth, and even blindness and cerebrovascular accidents (strokes). (Figure 12.18b).
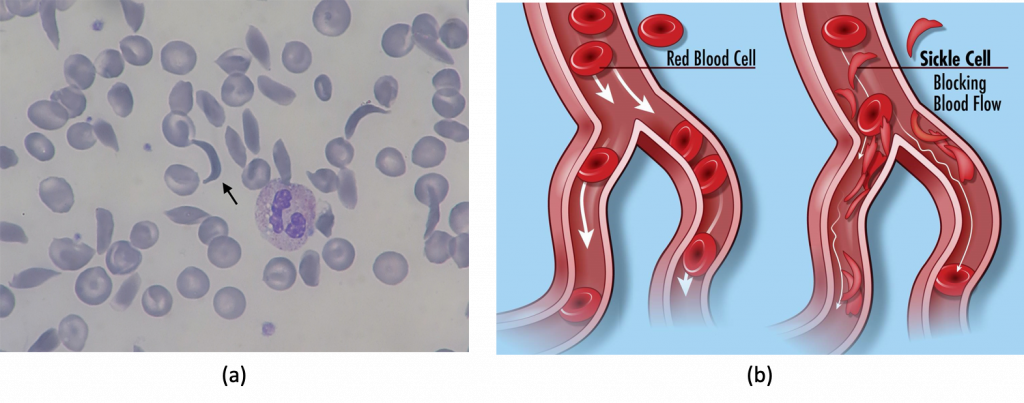
The normal allele and the sickle-cell allele are codominant, which means that both alleles are expressed. The genetics of the sickle-cell allele show that there are three genotypes and three phenotypes:
- The homozygous normal genotype (two normal hemoglobin alleles) results in a normal phenotype (all hemoglobin proteins are normal)
- The heterozygous genotype (one of each allele) results in a mild sickle-cell phenotype (half normal hemoglobin proteins and half non-functional hemoglobin proteins).
- The homozygous sickle-cell genotype (two sickle-cell alleles) results in a full-blown sickle-cell phenotype (all the hemoglobin proteins are non-functional).
When two individuals are heterozygous and have mild sickle-cell anemia, they have a 25% chance of having a child with full-blown sickle-cell anemia and a 50% chance of having a child with mild sickle-cell anemia (Figure 12.19).
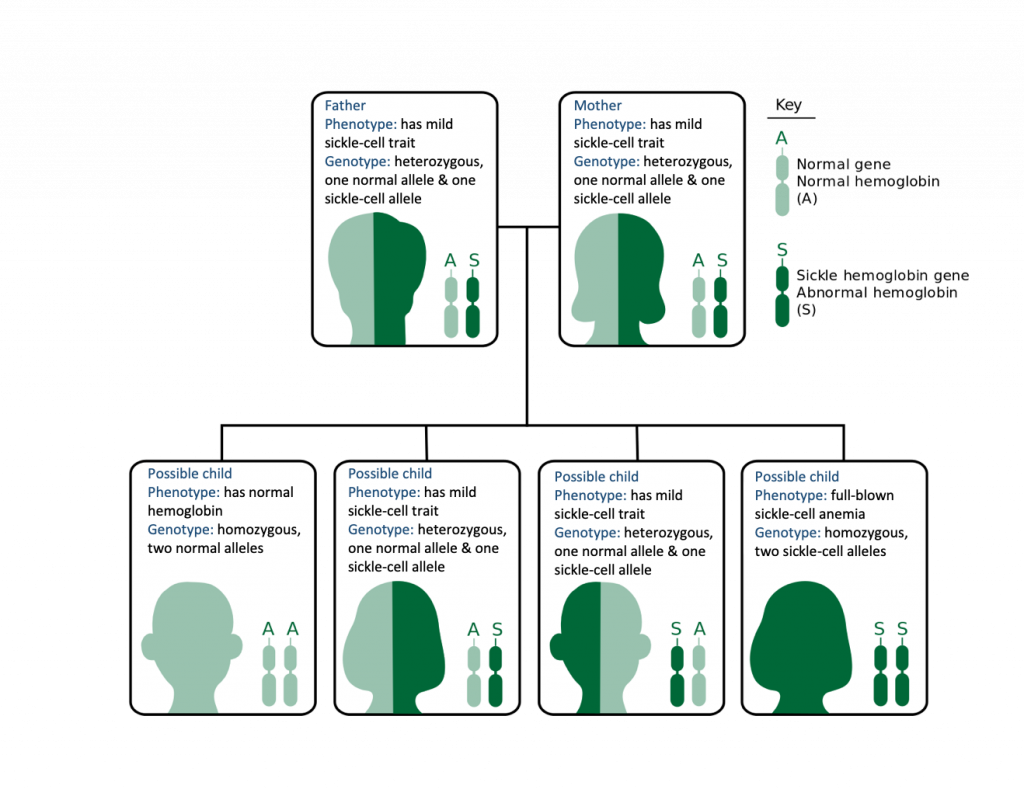
Sickle-cell anemia is a very serious disease. People with full-blown sickle-cell anemia rarely live to adulthood. People with the mild sickle-cell trait (heterozygous) suffer from an assortment of health problems and painful sickling attacks. It would seem as if this mutated allele for the hemoglobin gene would have been selected against shortly after the mutation first arose. Individuals with full-blown sickle-cell anemia rarely have children and people with the mild sickle-cell trait have difficulties having children. Yet, sickle-cell anemia is still fairly common in some human populations.
To understand why sickle-cell anemia is common in some human populations, it is important to examine the environmental context of the recent ancestors of those populations. For example, the sickle-cell allele is found in populations in West Africa and populations with recent ancestors from West Africa, such as African Americans. The environment of West Africa is plagued by a deadly blood parasite called malaria. People are infected when they are bitten by a mosquito that carries the parasite. The mosquito obtained the malarial parasite by first biting another individual who is already infected with malaria (Figure 12.20). The infection causes a serious disease characterized by high fevers, chills, nausea and vomiting, and muscle pain and fatigue. Malaria is one of the leading causes of death in the world (approximately half a million deaths every year), with almost 90% of the deaths occurring in Africa[3]. The most vulnerable individuals, i.e. the most likely to die from infection of the malarial parasite, are under the age of five.
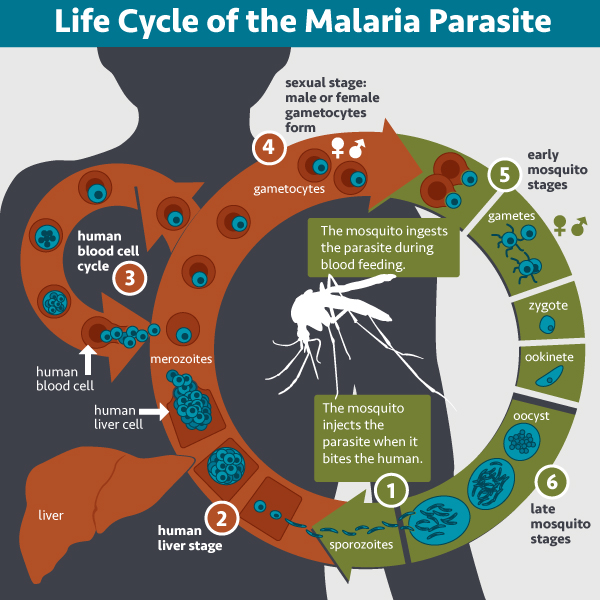
However, the malarial parasite has difficulty infecting sickled red blood cells. People with either full-blown or mild sickle-cell anemia are less likely to become infected and less likely to die of malaria. This creates a slight selective pressure for the sickle-cell allele in areas where malaria is common (Figure 12.21).
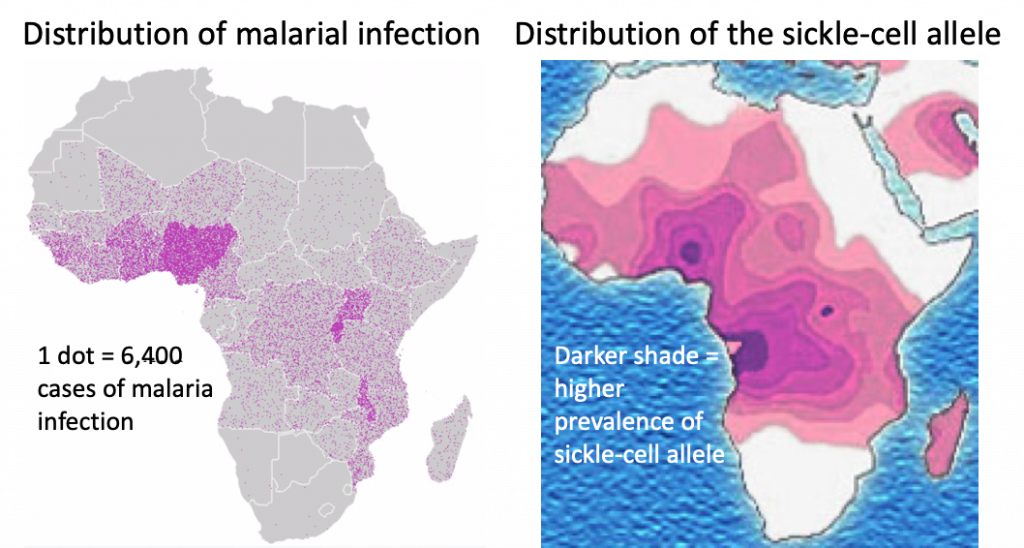
Reexamining the possible genotypes and phenotypes in a malarial context makes the selection for the sickle-cell allele evident. However, there is a tragic trade-off. Someone who has normal hemoglobin is selected against because they are more likely to die of malaria. Someone who has full-blown sickle-cell anemia is much more likely to die of the genetic disease. Someone who has mild sickle-cell anemia (heterozygotes) are less likely to die of malaria and only a slight chance of dying from sickle-cell anemia. In a malarial environment, if two individuals with this balanced genotype have children, only 50% of the children will have both selective advantages: lowered risk of dying from malaria and lowered risk of dying of sickle-cell anemia (Figure 12.22).
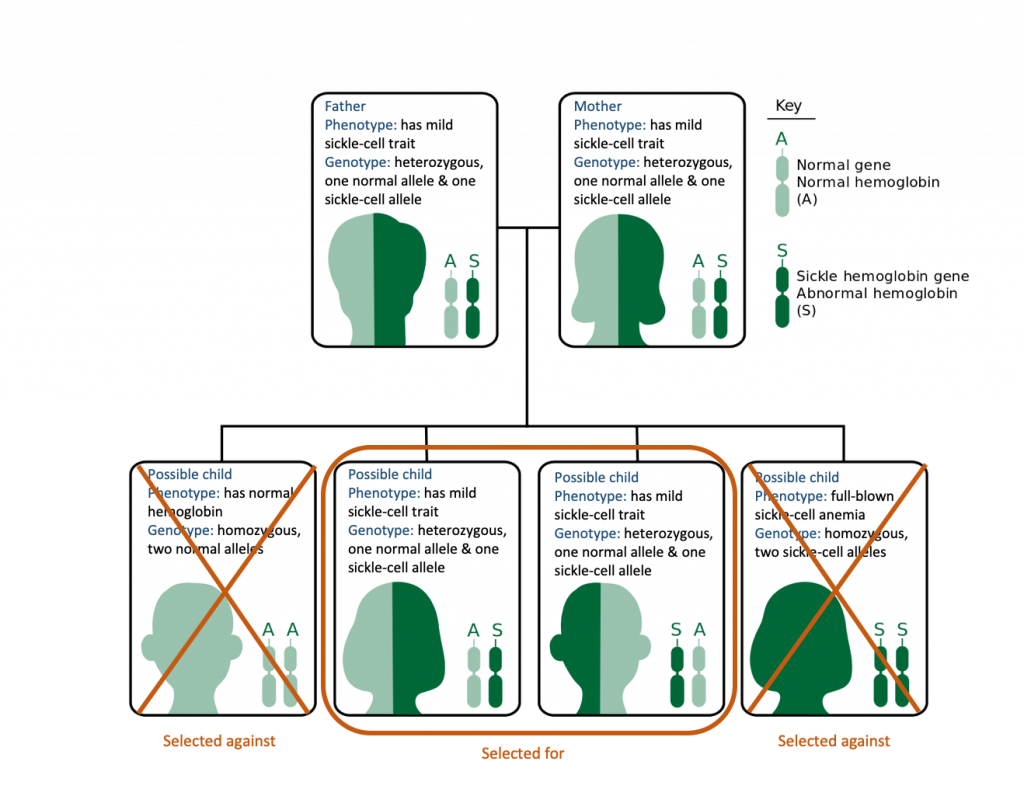
12.3 Other Mechanisms of Evolution
The four most important evolutionary forces that will change a population of organisms over time are natural selection, mutation, genetic drift, and migration or gene flow into or out of a population. Natural selection is described in detail in the preceding section. This section will cover the remainder of mechanisms of evolution.
12.3.1 Mutation: the source of all new variation
Mutation is a change in a DNA sequence. It can be a small alteration, such as a single base-pair change, through more extensive changes such as the addition or deletion of a base-pairs, though large alterations at a chromosomal level. Even small changes can make large differences in the protein that the DNA codes for, as seem above in the example of sickle cell anemia.
Mutation transforms one allele into a new allele. That new allele has a very small frequency during the first generation. Over several generations, its frequency will slowly increase in a population if no other evolutionary forces act on the allele. If natural selection acts against the allele, it will be removed from the population. This is one reason that genetic diseases remain in the human population at very low frequencies. If the allele is favored by selection, it will increase in frequency. If the mutation is neutral – neither increases or decreases reproductive fitness – its frequency will remain static in a population for generations.
12.3.2. Gene Flow/Migration
If two populations of a species have different allele frequencies, migration of individuals from one population to another them will cause frequency changes in both populations. The new population will have an increase in certain alleles while the previous population will experience a decrease in certain alleles (Figure 12.23).
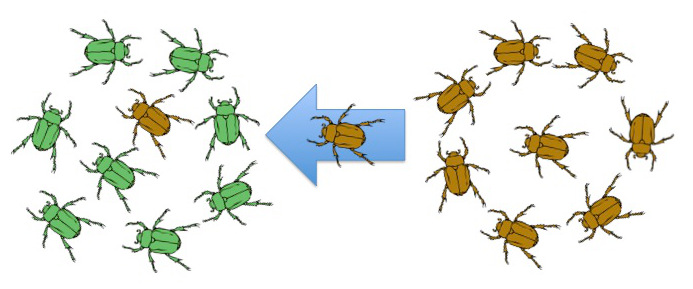
After migrating, the new individual(s) mates with local individuals and the novel allele(s) becomes part of the population’s gene pool.
While some populations are fairly stable, others experience more flux. Some animals do not travel widely during their lifetime whereas some species are highly migratory and gene flow is very common. Plant species may seem very fixed in their location, but they also experience gene flow. Many plants can send their seeds far and wide by wind or in the guts of animals who have eaten the seeds and then deposit them in a different location when they defecate. These widely traveling seeds may introduce alleles common in the source population to a new population in which they are rare.
12.3.3 Genetic Drift
Another way a population’s allele frequencies can change is genetic drift (Figure 11.7), which is simply the effect of chance. Genetic drift is most important in small populations. Drift would be completely absent in a population with infinite individuals, but, of course, no population is this large. Genetic drift occurs because the alleles in an offspring generation are a random sample of the alleles in the parent generation. Alleles may or may not make it into the next generation due to chance events including mortality of an individual, events affecting finding a mate, and even the events affecting which gametes end up in fertilizations. If one individual in a population of ten individuals happens to die before it leaves any offspring to the next generation, all of its genes—a tenth of the population’s gene pool—will be suddenly lost. In a population of 100, that 1 individual represents only 1 percent of the overall gene pool; therefore, it has much less impact on the population’s genetic structure and is unlikely to remove all copies of even a relatively rare allele.
Imagine a population of ten individuals, half with allele A and half with allele a (the individuals are haploid). In a stable population, the next generation will also have ten individuals. Choose that generation randomly by flipping a coin ten times and let heads be A and tails be a. It is unlikely that the next generation will have exactly half of each allele. There might be six of one and four of the other, or some different set of frequencies. Thus, the allele frequencies have changed and evolution has occurred. A coin will no longer work to choose the next generation (because the odds are no longer one half for each allele). The frequency in each generation will drift up and down on what is known as a random walk until at one point either all A or all a are chosen and that allele is fixed from that point on. This could take a very long time for a large population. This simplification is not very biological, but it can be shown that real populations behave this way. The effect of drift on frequencies is greater the smaller a population is. Its effect is also greater on an allele with a frequency far from one half. Drift will influence every allele, even those that are being naturally selected.
Genetic drift can also be magnified by natural or human-caused events, such as a disaster that randomly kills a large portion of the population, which is known as the bottleneck effect that results in a large portion of the genome suddenly being wiped out (Figure 11.8). In one fell swoop, the genetic structure of the survivors becomes the genetic structure of the entire population, which may be very different from the pre-disaster population. The disaster must be one that kills for reasons unrelated to the organism’s traits, such as a hurricane or lava flow. A mass killing caused by unusually cold temperatures at night, is likely to affect individuals differently depending on the alleles they possess that confer cold hardiness.
Another scenario in which populations might experience a strong influence of genetic drift is if some portion of the population leaves to start a new population in a new location, or if a population gets divided by a physical barrier of some kind. In this situation, those individuals are unlikely to be representative of the entire population which results in the founder effect. The founder effect occurs when the genetic structure matches that of the new population’s founding fathers and mothers. The founder effect is believed to have been a key factor in the genetic history of the Afrikaner population of Dutch settlers in South Africa, as evidenced by mutations that are common in Afrikaners but rare in most other populations. This is likely due to a higher-than-normal proportion of the founding colonists, which were a small sample of the original population, carried these mutations. As a result, the population expresses unusually high incidences of Huntington’s disease (HD) and Fanconi anemia (FA), a genetic disorder known to cause bone marrow and congenital abnormalities, and even cancer.
Media Attributions
- 11.1 diversity of life
- 11.10 horse fossil record
- homology limbs
- blue whale pelvis © Travis S. is licensed under a CC BY-NC (Attribution NonCommercial) license
- 11.12 arctic fox ptarmigan plumage
- Ernst Haekel embryos adapted by Nancy Barrickman is licensed under a CC BY (Attribution) license
- DNA great apes © Dave Huth is licensed under a CC BY (Attribution) license
- 11.13 proteacea plants
- galapagos islands © Antti Liponen is licensed under a CC BY (Attribution) license
- 11.2 Darwin finches
- desert adaptations
- competition hyena lion © Megan Coughlin is licensed under a CC BY-ND (Attribution NoDerivatives) license
- differential reproductive success © Nancy Barrickman
- natural selection summary © Nancy Barrickman
- 11.4 Grant finches
- 11.6 peppered moth
- sickle cell smear and blockage
- sickle cell genotypes and phenotypes © University of Michigan Medical School
- malaria life cycle © NIAID is licensed under a CC BY (Attribution) license
- sickle cell and malaria distribution
- sickle cell selection © Akiyao from the University of Michigan Medical School is licensed under a CC BY-SA (Attribution ShareAlike) license
- Gene Flow © Tsaneda is licensed under a CC BY (Attribution) license
- Theodosius Dobzhansky. “Biology, Molecular and Organismic.” American Zoologist 4, no. 4 (1964): 449. ↵
- Charles Darwin, Journal of Researches into the Natural History and Geology of the Countries Visited during the Voyage of H.M.S. Beagle Round the World, under the Command of Capt. Fitz Roy, R.N, 2nd. ed. (London: John Murray, 1860), http://www.archive.org/details/journalofresea00darw. ↵
- From "Malaria's World Impact" by the Centers for Disease Control, https://www.cdc.gov/malaria/malaria_worldwide/impact.html, accessed August 4, 2020 ↵