5 Chapter 5: Metabolism, Cellular Respiration and Photosynthesis
Chapter Outline
- Energy and Metabolism
- ATP: Adenosine Triphosphate
- Enzymes
- Energy in Living System
- Glycolysis
- Oxidation of Pyruvate and the Citric Acid Cycle
- Oxidative Phosphorylation
- Metabolism Without Oxygen
- Connections of Carbohydrates, Proteins, and Lipid Metabolic Pathways
- Regulation of Cellular Respiration
- Overview of Photosynthesis
- The Light-Dependent Reactions of Photosynthesis
- Using Light Energy to Make Organic Molecules
Figure 5.1 A hummingbird needs energy to maintain prolonged periods of flight. The bird obtains its energy from taking in food and transforming the nutrients into energy through a series of biochemical reactions. The flight muscles in birds are extremely efficient in energy production.(credit: modification of work by Cory Zanker)
Introduction
Virtually every task performed by living organisms requires energy. Energy is needed to perform heavy labor and exercise, but humans also use a great deal of energy while thinking, and even during sleep. In fact, the living cells of every organism constantly use energy. Nutrients and other molecules are imported, metabolized (broken down) and possibly synthesized into new molecules, modified if needed, transported around the cell, and may be distributed to the entire organism. For example, the large proteins that make up muscles are actively built from smaller molecules. Complex carbohydrates are broken down into simple sugars that the cell uses for energy. Just as energy is required to both build and demolish a building, energy is required for both the synthesis and breakdown of molecules. Additionally, signaling molecules such as hormones and neurotransmitters are transported between cells. Pathogenic bacteria and viruses are ingested and broken down by cells. Cells must also export waste and toxins to stay healthy, and many cells must swim or move surrounding materials via the beating motion of cellular appendages like cilia and flagella.
The cellular processes listed above require a steady supply of energy. From where, and in what form, does this energy come? How do living cells obtain energy, and how do they use it? This chapter will discuss different forms of energy and the physical laws that govern energy transfer. This chapter will also describe how cells use energy and replenish it, and how chemical reactions in the cell are performed with great efficiency.
Learning Objectives
You will be able to describe these basic functions of metabolism:
- define metabolism
- describe the role of enzymes in metabolism
- recognize that enzymes are proteins
- describe the role of ATP in cellular processes
- understand energy production in a cell from sources like glucose
- understand ATP production from light in photosynthesis
5.1 | Energy and Metabolism
Scientists use the term bioenergetics to discuss the concept of energy flow (Figure 5.2) through living systems, such as cells. Cellular processes such as the building and breaking down of complex molecules occur through stepwise chemical reactions. Some of these chemical reactions are spontaneous and release energy, whereas others require energy to proceed. Just as living things must continually consume food to replenish what has been used, cells must continually produce more energy to replenish that used by the many energy-requiring chemical reactions that constantly take place. All of the chemical reactions that take place inside cells, including those that use energy and those that release energy, are the cell’s metabolism.
Figure 5.2 Most life forms on earth get their energy from the sun. Plants use photosynthesis to capture sunlight, and herbivores eat those plants to obtain energy. Carnivores eat the herbivores, and decomposers digest plant and animal matter.
Metabolism of Carbohydrates
The metabolism of sugar (a simple carbohydrate) is a classic example of the many cellular processes that use and produce energy. Living things consume sugar as a major energy source, because sugar molecules have a great deal of energy stored within their bonds. The breakdown of glucose, a simple sugar, is described by the equation:
C6H12O6 + 6O2 → 6CO2 + 6H2O + energy
Carbohydrates that are consumed have their origins in photosynthesizing organisms like plants (Figure 5.3). During photosynthesis, plants use the energy of sunlight to convert carbon dioxide gas (CO2) into sugar molecules, like glucose (C6H12O6). Because this process involves synthesizing a larger, energy storing molecule, it requires an input of energy to proceed. The synthesis of glucose is described by this equation (notice that it is the reverse of the previous equation):
6CO2 + 6H2O + energy → C6H12O6 + 6O2
During the chemical reactions of photosynthesis, energy is provided in the form of a very high-energy molecule called ATP, or adenosine triphosphate, which is the primary energy currency of all cells. Just as the dollar is used as currency to buy goods, cells use molecules of ATP as energy currency to perform immediate work. The sugar (glucose) is stored as starch or glycogen. Energy-storing polymers like these are broken down into glucose to supply molecules of ATP.
Solar energy is required to synthesize a molecule of glucose during the reactions of photosynthesis. In photosynthesis, light energy from the sun is initially transformed into chemical energy that is temporally stored in the energy carrier molecules ATP and NADPH (nicotinamide adenine dinucleotide phosphate). The stored energy in ATP and NADPH is then used later in photosynthesis to build one molecule of glucose from six molecules of CO2. This process is analogous to eating breakfast in the morning to acquire energy for your body that can be used later in the day. Under ideal conditions, energy from 18 molecules of ATP is required to synthesize one molecule of glucose during the reactions of photosynthesis. Glucose molecules can also be combined with and converted into other types of sugars. When sugars are consumed, molecules of glucose eventually make their way into each living cell of the organism. Inside the cell, each sugar molecule is broken down through a complex series of chemical reactions. The goal of these reactions is to harvest the energy stored inside the sugar molecules. The harvested energy is used to make high-energy ATP molecules, which can be used to perform work, powering many chemical reactions in the cell. The amount of energy needed to make one molecule of glucose from six molecules of carbon dioxide is 18 molecules of ATP and 12 molecules of NADPH (each one of which is energetically equivalent to three molecules of ATP), or a total of 54 molecule equivalents required for the synthesis of one molecule of glucose. This process is a fundamental and efficient way for cells to generate the molecular energy that they require.
Figure 5.3 Plants, like this oak tree and acorn, use energy from sunlight to make sugar and other organic molecules. Both plants and animals (like this squirrel) use cellular respiration to derive energy from the organic molecules originally produced by plants. (credit “acorn”: modification of work by Noel Reynolds; credit “squirrel”: modification of work by Dawn Huczek)
Metabolic Pathways
The processes of making and breaking down sugar molecules illustrate two types of metabolic pathways. A metabolic pathway is a series of interconnected biochemical reactions that convert a substrate molecule or molecules, step-by-step, through a series of metabolic intermediates, eventually yielding a final product or products. In the case of sugar metabolism, the first metabolic pathway synthesized sugar from smaller molecules, and the other pathway broke sugar down into smaller molecules. These two opposite processes—the first requiring energy and the second producing energy—are referred to as anabolic (building) and catabolic (breaking down) pathways, respectively. Consequently, metabolism is composed of building (anabolism) and degradation (catabolism).
Anabolic and Catabolic Pathways
Anabolic pathways require an input of energy to synthesize complex molecules from simpler ones. Synthesizing sugar from CO2 is one example. Other examples are the synthesis of large proteins from amino acid building blocks, and the synthesis of new DNA strands from nucleic acid building blocks. These biosynthetic processes are critical to the life of the cell, take place constantly, and demand energy provided by ATP and other high-energy molecules like NADH (nicotinamide adenine dinucleotide) and NADPH (Figure 5.5).
It is important to know that the chemical reactions of metabolic pathways don’t take place spontaneously. Each reaction step is facilitated, or catalyzed, by a protein called an enzyme. Enzymes are important for catalyzing all types of biological reactions—those that require energy as well as those that release energy.
Figure 5.5 Anabolic pathways are those that require energy to synthesize larger molecules. Catabolic pathways are those that generate energy by breaking down larger molecules. Both types of pathways are required for maintaining the cell’s energy balance.
5.2 | ATP: Adenosine Triphosphate
Even exergonic, energy-releasing reactions require a small amount of activation energy in order to proceed. However, consider endergonic reactions, which require much more energy input, because their products have more free energy than their reactants. Within the cell, where does energy to power such reactions come from? The answer lies with an energy-supplying molecule called adenosine triphosphate, or ATP. ATP is a small, relatively simple molecule (Figure 5.13), but within some of its bonds, it contains the potential for a quick burst of energy that can be harnessed to perform cellular work. This molecule can be thought of as the primary energy currency of cells in much the same way that money is the currency that people exchange for things they need. ATP is used to power the majority of energy-requiring cellular reactions.
Figure 5.13 ATP is the primary energy currency of the cell. It has an adenosine backbone with three phosphate groups attached.
As its name suggests, adenosine triphosphate is comprised of adenosine bound to three phosphate groups (Figure 5.13). Adenosine is a nucleoside consisting of the nitrogenous base adenine and a five-carbon sugar, ribose. The three phosphate groups, in order of closest to furthest from the ribose sugar, are labeled alpha, beta, and gamma. Together, these chemical groups constitute an energy powerhouse. However, not all bonds within this molecule exist in a particularly high-energy state. Both bonds that link the phosphates are equally high-energy bonds (phosphoanhydride bonds) that, when broken, release sufficient energy to power a variety of cellular reactions and processes. These high-energy bonds are the bonds between the second and third (or beta and gamma) phosphate groups and between the first and second phosphate groups. The reason that these bonds are considered “high-energy” is because the products of such bond breaking—adenosine diphosphate (ADP) and one inorganic phosphate group (Pi)—have considerably lower free energy than the reactants: ATP and a water molecule. Because this reaction takes place with the use of a water molecule, it is considered a hydrolysis reaction. In other words, ATP is hydrolyzed into ADP in the following reaction:
ATP + H2O → ADP + Pi + free energy
Like most chemical reactions, the hydrolysis of ATP to ADP is reversible. The reverse reaction regenerates ATP from ADP + Pi. Indeed, cells rely on the regeneration of ATP just as people rely on the regeneration of spent money through some sort of income. Since ATP hydrolysis releases energy, ATP regeneration must require an input of free energy. The formation of ATP is expressed in this equation:
ADP + Pi + free energy → ATP + H2O
Two prominent questions remain with regard to the use of ATP as an energy source. Exactly how much free energy is released with the hydrolysis of ATP, and how is that free energy used to do cellular work? The calculated ∆G for the hydrolysis of one mole of ATP into ADP and Pi is −7.3 kcal/mole (−30.5 kJ/ mol). Since this calculation is true under standard conditions, it would be expected that a different value exists under cellular conditions. In fact, the ∆G for the hydrolysis of one mole of ATP in a living cell is almost double the value at standard conditions: 14 kcal/mol (−57 kJ/mol).
ATP is a highly unstable molecule. Unless quickly used to perform work, ATP spontaneously dissociates into ADP + Pi, and the free energy released during this process is lost as heat. The second question posed above, that is, how the energy released by ATP hydrolysis is used to perform work inside the cell, depends on a strategy called energy coupling. Cells couple the exergonic reaction of ATP hydrolysis with endergonic reactions, allowing them to proceed. One example of energy coupling using ATP involves a transmembrane ion pump that is extremely important for cellular function. This sodium-potassium pump (Na+/K+ pump) drives sodium out of the cell and potassium into the cell (Figure 5.14). A large percentage of a cell’s ATP is spent powering this pump, because cellular processes bring a great deal of sodium into the cell and potassium out of the cell. The pump works constantly to stabilize cellular concentrations of sodium and potassium. In order for the pump to turn one cycle (exporting three Na+ ions and importing two K+ ions), one molecule of ATP must be hydrolyzed. When ATP is hydrolyzed, its gamma phosphate doesn’t simply float away, but is actually transferred onto the pump protein. This process of a phosphate group binding to a molecule is called phosphorylation. As with most cases of ATP hydrolysis, a phosphate from ATP is transferred onto another molecule. In a phosphorylated state, the Na+/K+ pump has more free energy and is triggered to undergo a conformational change. This change allows it to release Na+ to the outside of the cell. It then binds extracellular K+, which, through another conformational change, causes the phosphate to detach from the pump. This release of phosphate triggers the K+ to be released to the inside of the cell. Essentially, the energy released from the hydrolysis of ATP is coupled with the energy required to power the pump and transport Na+ and K+ ions. ATP performs cellular work using this basic form of energy coupling through phosphorylation.
Often during cellular metabolic reactions, such as the synthesis and breakdown of nutrients, certain molecules must be altered slightly in their conformation to become substrates for the next step in the reaction series. One example is during the very first steps of cellular respiration, when a molecule of the sugar glucose is broken down in the process of glycolysis. In the first step of this process, ATP is required for the phosphorylation of glucose, creating a high-energy but unstable intermediate. This phosphorylation reaction powers a conformational change that allows the phosphorylated glucose molecule to be converted to the phosphorylated sugar fructose. Fructose is a necessary intermediate for glycolysis to move forward. Here, the exergonic reaction of ATP hydrolysis is coupled with the endergonic reaction of converting glucose into a phosphorylated intermediate in the pathway. Once again, the energy released by breaking a phosphate bond within ATP was used for the phosphorylation of another molecule, creating an unstable intermediate and powering an important conformational change.
See an interactive animation of the ATP-producing glycolysis process at this site(http://openstaxcollege.org/l/glycolysis_stgs) .
5.3 | Enzymes
A substance that helps a chemical reaction to occur is a catalyst, and the special molecules that catalyze biochemical reactions are called enzymes. Almost all enzymes are proteins, made up of chains of amino acids, and they perform the critical task of lowering the activation energies of chemical reactions inside the cell. Enzymes do this by binding to the reactant molecules, and holding them in such a way as to make the chemical bond-breaking and bond-forming processes take place more readily. It is important to remember that enzymes don’t change the ∆G of a reaction. In other words, they don’t change whether a reaction is exergonic (spontaneous) or endergonic. This is because they don’t change the free energy of the reactants or products. They only reduce the activation energy required to reach the transition state (Figure 5.15).
Figure 5.15 Enzymes lower the activation energy of the reaction but do not change the free energy of the reaction.
Enzyme Active Site and Substrate Specificity
The chemical reactants to which an enzyme binds are the enzyme’s substrates. There may be one or more substrates, depending on the particular chemical reaction. In some reactions, a single-reactant substrate is broken down into multiple products. In others, two substrates may come together to create one larger molecule. Two reactants might also enter a reaction, both become modified, and leave the reaction as two products. The location within the enzyme where the substrate binds is called the enzyme’s active site. The active site is where the “action” happens, so to speak. Since enzymes are proteins, there is a unique combination of amino acid residues (also called side chains, or R groups) within the active site. Each residue is characterized by different properties. Residues can be large or small, weakly acidic or basic, hydrophilic or hydrophobic, positively or negatively charged, or neutral. The unique combination of amino acid residues, their positions, sequences, structures, and properties, creates a very specific chemical environment within the active site. This specific environment is suited to bind, albeit briefly, to a specific chemical substrate (or substrates). Due to this jigsaw puzzle-like match between an enzyme and its substrates (which adapts to find the best fit between the transition state and the active site), enzymes are known for their specificity. The “best fit” results from the shape and the amino acid functional group’s attraction to the substrate. There is a specifically matched enzyme for each substrate and, thus, for each chemical reaction; however, there is flexibility as well.
The fact that active sites are so perfectly suited to provide specific environmental conditions also means that they are subject to influences by the local environment. It is true that increasing the environmental temperature generally increases reaction rates, enzyme-catalyzed or otherwise. However, increasing or decreasing the temperature outside of an optimal range can affect chemical bonds within the active site in such a way that they are less well suited to bind substrates. High temperatures will eventually cause enzymes, like other biological molecules, to denature, a process that changes the natural properties of a substance. Likewise, the pH of the local environment can also affect enzyme function. Active site amino acid residues have their own acidic or basic properties that are optimal for catalysis. These residues are sensitive to changes in pH that can impair the way substrate molecules bind. Enzymes are suited to function best within a certain pH range, and, as with temperature, extreme pH values (acidic or basic) of the environment can cause enzymes to denature.
Induced Fit and Enzyme Function
For many years, scientists thought that enzyme-substrate binding took place in a simple “lock-and-key” fashion. This model asserted that the enzyme and substrate fit together perfectly in one instantaneous step. However, current research supports a more refined view called induced fit (Figure 5.16). The induced-fit model expands upon the lock-and-key model by describing a more dynamic interaction between enzyme and substrate. As the enzyme and substrate come together, their interaction causes a mild shift in the enzyme’s structure that confirms an ideal binding arrangement between the enzyme and the transition state of the substrate. This ideal binding maximizes the enzyme’s ability to catalyze its reaction.
View an animation of induced fit at this website (http://openstaxcollege.org/l/hexokinase) .
You’ve learned that the activation energy required for many reactions includes the energy involved in manipulating or slightly contorting chemical bonds so that they can easily break and allow others to reform. Enzymatic action can aid this process. The enzyme-substrate complex can lower the activation energy by contorting substrate molecules in such a way as to facilitate bond-breaking, helping to reach the transition state. Finally, enzymes can also lower activation energies by taking part in the chemical reaction itself. The amino acid residues can provide certain ions or chemical groups that actually form covalent bonds with substrate molecules as a necessary step of the reaction process. In these cases, it is important to remember that the enzyme will always return to its original state at the completion of the reaction. One of the hallmark properties of enzymes is that they remain ultimately unchanged by the reactions they catalyze. After an enzyme is done catalyzing a reaction, it releases its product(s).
Figure 5.16 According to the induced-fit model, both enzyme and substrate undergo dynamic conformational changes upon binding. The enzyme contorts the substrate into its transition state, thereby increasing the rate of the reaction.
Control of Metabolism Through Enzyme Regulation
It would seem ideal to have a scenario in which all of the enzymes encoded in an organism’s genome existed in abundant supply and functioned optimally under all cellular conditions, in all cells, at all times. In reality, this is far from the case. A variety of mechanisms ensure that this does not happen. Cellular needs and conditions vary from cell to cell, and change within individual cells over time. The required enzymes and energetic demands of stomach cells are different from those of fat storage cells, skin cells, blood cells, and nerve cells. Furthermore, a digestive cell works much harder to process and break down nutrients during the time that closely follows a meal compared with many hours after a meal. As these cellular demands and conditions vary, so do the amounts and functionality of different enzymes.
Regulation of Enzymes by Molecules
Enzymes can be regulated in ways that either promote or reduce their activity. There are many different kinds of molecules that inhibit or promote enzyme function, and various mechanisms exist for doing so. In some cases of enzyme inhibition, for example, an inhibitor molecule is similar enough to a substrate that it can bind to the active site and simply block the substrate from binding. When this happens, the enzyme is inhibited through competitive inhibition, because an inhibitor molecule competes with the substrate for active site binding (Figure 5.17). On the other hand, in noncompetitive inhibition, an inhibitor molecule binds to the enzyme in a location other than an allosteric site and still manages to block substrate binding to the active site.
Figure 5.17 Competitive and noncompetitive inhibition affect the rate of reaction differently. Competitive inhibitors affect the initial rate but do not affect the maximal rate, whereas noncompetitive inhibitors affect the maximal rate.
Some inhibitor molecules bind to enzymes in a location where their binding induces a conformational change that reduces the affinity of the enzyme for its substrate. This type of inhibition is called allosteric inhibition (Figure 5.18). Most allosterically regulated enzymes are made up of more than one polypeptide, meaning that they have more than one protein subunit. When an allosteric inhibitor binds to an enzyme, all active sites on the protein subunits are changed slightly such that they bind their substrates with less efficiency. There are allosteric activators as well as inhibitors. Allosteric activators bind to locations on an enzyme away from the active site, inducing a conformational change that increases the affinity of the enzyme’s active site(s) for its substrate(s).
Figure 5.18 Allosteric inhibitors modify the active site of the enzyme so that substrate binding is reduced or prevented. In contrast, allosteric activators modify the active site of the enzyme so that the affinity for the substrate increases.
Many enzymes don’t work optimally, or even at all, unless bound to other specific non-protein helper molecules, either temporarily through ionic or hydrogen bonds or permanently through stronger covalent bonds. Two types of helper molecules are cofactors and coenzymes. Binding to these molecules promotes optimal conformation and function for their respective enzymes. Cofactors are inorganic ions such as iron (Fe++) and magnesium (Mg++). One example of an enzyme that requires a metal ion as a cofactor is the enzyme that builds DNA molecules, DNA polymerase, which requires bound zinc ion (Zn++) to function. Coenzymes are organic helper molecules, with a basic atomic structure made up of carbon and hydrogen, which are required for enzyme action. The most common sources of coenzymes are dietary vitamins (Figure 5.20). Some vitamins are precursors to coenzymes and others act directly as coenzymes. Vitamin C is a coenzyme for multiple enzymes that take part in building the important connective tissue component, collagen. An important step in the breakdown of glucose to yield energy is catalysis by a multi-enzyme complex called pyruvate dehydrogenase. Pyruvate dehydrogenase is a complex of several enzymes that actually requires one cofactor (a magnesium ion) and five different organic coenzymes to catalyze its specific chemical reaction. Therefore, enzyme function is, in part, regulated by an abundance of various cofactors and coenzymes, which are supplied primarily by the diets of most organisms.
Figure 5.20 Vitamins are important coenzymes or precursors of coenzymes, and are required for enzymes to function properly. Multivitamin capsules usually contain mixtures of all the vitamins at different percentages.
Enzyme Compartmentalization
In eukaryotic cells, molecules such as enzymes are usually compartmentalized into different organelles. This allows for yet another level of regulation of enzyme activity. Enzymes required only for certain cellular processes can be housed separately along with their substrates, allowing for more efficient chemical reactions. Examples of this sort of enzyme regulation based on location and proximity include the enzymes involved in the latter stages of cellular respiration, which take place exclusively in the mitochondria, and the enzymes involved in the digestion of cellular debris and foreign materials, located within lysosomes.
Feedback Inhibition in Metabolic Pathways
Molecules can regulate enzyme function in many ways. A major question remains, however: What are these molecules and where do they come from? Some are cofactors and coenzymes, ions, and organic molecules, as you’ve learned. What other molecules in the cell provide enzymatic regulation, such as allosteric modulation, and competitive and noncompetitive inhibition? The answer is that a wide variety of molecules can perform these roles. Some of these molecules include pharmaceutical and non-pharmaceutical drugs, toxins, and poisons from the environment. Perhaps the most relevant sources of enzyme regulatory molecules, with respect to cellular metabolism, are the products of the cellular metabolic reactions themselves. In a most efficient and elegant way, cells have evolved to use the products of their own reactions for feedback inhibition of enzyme activity. Feedback inhibition involves the use of a reaction product to regulate its own further production (Figure 5.21). The cell responds to the abundance of specific products by slowing down production during anabolic or catabolic reactions. Such reaction products may inhibit the enzymes that catalyzed their production through the mechanisms described above.
Figure 5.21 Metabolic pathways are a series of reactions catalyzed by multiple enzymes. Feedback inhibition, where the end product of the pathway inhibits an upstream step, is an important regulatory mechanism in cells.
The production of both amino acids and nucleotides is controlled through feedback inhibition. Additionally, ATP is an allosteric regulator of some of the enzymes involved in the catabolic breakdown of sugar, the process that produces ATP. In this way, when ATP is abundant, the cell can prevent its further production. Remember that ATP is an unstable molecule that can spontaneously dissociate into ADP. If too much ATP were present in a cell, much of it would go to waste. On the other hand, ADP serves as a positive allosteric regulator (an allosteric activator) for some of the same enzymes that are inhibited by ATP. Thus, when relative levels of ADP are high compared to ATP, the cell is triggered to produce more ATP through the catabolism of sugar.
5.4 | Energy in Living Systems
Energy production within a cell involves many coordinated chemical pathways. Most of these pathways are combinations of oxidation and reduction reactions. Oxidation and reduction occur in tandem. An oxidation reaction strips an electron from an atom in a compound, and the addition of this electron to another compound is a reduction reaction. Because oxidation and reduction usually occur together, these pairs of reactions are called oxidation reduction reactions, or redox reactions.
ATP in Living Systems
A living cell cannot store significant amounts of free energy. Excess free energy would result in an increase of heat in the cell, which would result in excessive thermal motion that could damage and then destroy the cell. Rather, a cell must be able to handle that energy in a way that enables the cell to store energy safely and release it for use only as needed. Living cells accomplish this by using the compound adenosine triphosphate (ATP). ATP is often called the “energy currency” of the cell, and, like currency, this versatile compound can be used to fill any energy need of the cell. How? It functions similarly to a rechargeable battery.
When ATP is broken down, usually by the removal of its terminal phosphate group, energy is released. The energy is used to do work by the cell, usually by the released phosphate binding to another molecule, activating it. For example, in the mechanical work of muscle contraction, ATP supplies the energy to move the contractile muscle proteins. Recall the active transport work of the sodium-potassium pump in cell membranes. ATP alters the structure of the integral protein that functions as the pump, changing its affinity for sodium and potassium. In this way, the cell performs work, pumping ions against their electrochemical gradients.
ATP Structure and Function
At the heart of ATP is a molecule of adenosine monophosphate (AMP), which is composed of an adenine molecule bonded to a ribose molecule and to a single phosphate group (Figure 5.22). Ribose is a five carbon sugar found in RNA, and AMP is one of the nucleotides in RNA. The addition of a second phosphate group to this core molecule results in the formation of adenosine diphosphate (ADP); the addition of a third phosphate group forms adenosine triphosphate (ATP).
Figure 5.22 ATP (adenosine triphosphate) has three phosphate groups that can be removed by hydrolysis to form ADP (adenosine diphosphate) or AMP (adenosine monophosphate).The negative charges on the phosphate group naturally repel each other, requiring energy to bond them together and releasing energy when these bonds are broken.
The addition of a phosphate group to a molecule requires energy. Phosphate groups are negatively charged and thus repel one another when they are arranged in series, as they are in ADP and ATP. This repulsion makes the ADP and ATP molecules inherently unstable. The release of one or two phosphate groups from ATP, a process called dephosphorylation, releases energy.
Energy from ATP
Hydrolysis is the process of breaking complex macromolecules apart. During hydrolysis, water is split, or lysed, and the resulting hydrogen atom (H+) and a hydroxyl group (OH–) are added to the larger molecule. The hydrolysis of ATP produces ADP, together with an inorganic phosphate ion (Pi), and the release of free energy. To carry out life processes, ATP is continuously broken down into ADP, and like a rechargeable battery, ADP is continuously regenerated into ATP by the reattachment of a third phosphate group. Water, which was broken down into its hydrogen atom and hydroxyl group during ATP hydrolysis, is regenerated when a third phosphate is added to the ADP molecule, reforming ATP.
Obviously, energy must be infused into the system to regenerate ATP. Where does this energy come from? In nearly every living thing on earth, the energy comes from the metabolism of glucose. In this way, ATP is a direct link between the limited set of exergonic pathways of glucose catabolism and the multitude of endergonic pathways that power living cells.
Phosphorylation
Recall that, in some chemical reactions, enzymes may bind to several substrates that react with each other on the enzyme, forming an intermediate complex. An intermediate complex is a temporary structure, and it allows one of the substrates (such as ATP) and reactants to more readily react with each other; in reactions involving ATP, ATP is one of the substrates and ADP is a product. During an endergonic chemical reaction, ATP forms an intermediate complex with the substrate and enzyme in the reaction. This intermediate complex allows the ATP to transfer its third phosphate group, with its energy, to the substrate, a process called phosphorylation. Phosphorylation refers to the addition of the phosphate (~P). This is illustrated by the following generic reaction:
A + enzyme + ATP → ⎡⎣A − enzyme − ∼ P⎤⎦ → B + enzyme + ADP + phosphate ion
When the intermediate complex breaks apart, the energy is used to modify the substrate and convert it into a product of the reaction. The ADP molecule and a free phosphate ion are released into the medium and are available for recycling through cell metabolism.
Substrate Phosphorylation
ATP is generated through two mechanisms during the breakdown of glucose. A few ATP molecules are generated (that is, regenerated from ADP) as a direct result of the chemical reactions that occur in the catabolic pathways. A phosphate group is removed from an intermediate reactant in the pathway, and the free energy of the reaction is used to add the third phosphate to an available ADP molecule, producing ATP (Figure 5.23). This very direct method of phosphorylation is called substrate-level phosphorylation.
Figure 5.23 In phosphorylation reactions, the gamma phosphate of ATP is attached to a protein.
Oxidative Phosphorylation
Most of the ATP generated during glucose catabolism, however, is derived from a much more complex process, chemiosmosis, which takes place in mitochondria (Figure 5.24) within a eukaryotic cell or the plasma membrane of a prokaryotic cell. Chemiosmosis, a process of ATP production in cellular metabolism, is used to generate 90 percent of the ATP made during glucose catabolism and is also the method used in the light reactions of photosynthesis to harness the energy of sunlight. The production of ATP using the process of chemiosmosis is called oxidative phosphorylation because of the involvement of oxygen in the process.
Figure 5.24 In eukaryotes, oxidative phosphorylation takes place in mitochondria. In prokaryotes, this process takes place in the plasma membrane. (Credit: modification of work by Mariana RuizVillareal)
5.5 | Glycolysis
You have read that nearly all of the energy used by living cells comes to them in the bonds of the sugar, glucose. Glycolysis is the first step in the breakdown of glucose to extract energy for cellular metabolism. Nearly all living organisms carry out glycolysis as part of their metabolism. The process does not use oxygen and is therefore anaerobic. Glycolysis takes place in the cytoplasm of both prokaryotic and eukaryotic cells. Glucose enters heterotrophic cells in two ways. One method is through secondary active transport in which the transport takes place against the glucose concentration gradient. The other mechanism uses a group of integral proteins called GLUT proteins, also known as glucose transporter proteins. These transporters assist in the facilitated diffusion of glucose.
Glycolysis begins with the six carbon ring-shaped structure of a single glucose molecule and ends with two molecules of a three-carbon sugar called pyruvate. Glycolysis consists of two distinct phases. The first part of the glycolysis pathway traps the glucose molecule in the cell and uses energy to modify it so that the six-carbon sugar molecule can be split evenly into the two three-carbon molecules. The second part of glycolysis extracts energy from the molecules and stores it in the form of ATP and NADH, the reduced form of NAD.
Figure 5.25 The first half of glycolysis uses two ATP molecules in the phosphorylation of glucose, which is then split into two three-carbon molecules.
Figure 5.26 The second half of glycolysis involves phosphorylation without ATP investment (step 6) and produces two NADH and four ATP molecules per glucose.
Gain a better understanding of the breakdown of glucose by glycolysis by visiting this site(http://openstaxcollege.org/l/glycolysis) to see the process in action.
Outcomes of Glycolysis
The last step in glycolysis will not occur if pyruvate kinase, the enzyme that catalyzes the formation of pyruvate, is not available in sufficient quantities. In this situation, the entire glycolysis pathway will proceed, but only two ATP molecules will be made in the second half. Thus, pyruvate kinase is a rate limiting enzyme for glycolysis.
5.6 | Oxidation of Pyruvate and the Citric Acid Cycle
If oxygen is available, aerobic respiration will go forward. In eukaryotic cells, the pyruvate molecules produced at the end of glycolysis are transported into mitochondria, which are the sites of cellular respiration. There, pyruvate will be transformed into an acetyl group that will be picked up and activated by a carrier compound called coenzyme A (CoA). The resulting compound is called acetyl CoA. CoA is made from vitamin B5, pantothenic acid. Acetyl CoA can be used in a variety of ways by the cell, but its major function is to deliver the acetyl group derived from pyruvate to the next stage of the pathway in glucose catabolism.
Breakdown of Pyruvate
In order for pyruvate, the product of glycolysis, to enter the next pathway, it must undergo several changes. The conversion is a three-step process (Figure 5.26).
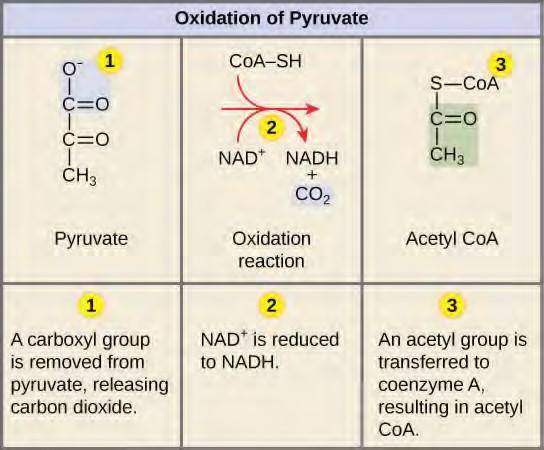
Note that during the second stage of glucose metabolism, whenever a carbon atom is removed, it is bound to two oxygen atoms, producing carbon dioxide, one of the major end products of cellular respiration.
Acetyl CoA to CO2
In the presence of oxygen, acetyl CoA delivers its acetyl group to a four-carbon molecule, oxaloacetate, to form citrate, a six-carbon molecule with three carboxyl groups; this pathway will harvest the remainder of the extractable energy from what began as a glucose molecule. This single pathway is called by different names: the citric acid cycle (for the first intermediate formed—citric acid, or citrate—when acetate joins to the oxaloacetate), the TCA cycle (since citric acid or citrate and isocitrate are tricarboxylic acids), and the Krebs cycle, after Hans Krebs, who first identified the steps in the pathway in the 1930s in pigeon flight muscles.
Citric Acid Cycle
Like the conversion of pyruvate to acetyl CoA, the citric acid cycle takes place in the matrix of mitochondria. Almost all of the enzymes of the citric acid cycle are soluble, with the single exception of the enzyme succinate dehydrogenase, which is embedded in the inner membrane of the mitochondrion. Unlike glycolysis, the citric acid cycle is a closed loop: The last part of the pathway regenerates the compound used in the first step. The eight steps of the cycle are a series of redox, dehydration, hydration, and decarboxylation reactions that produce two carbon dioxide molecules, one GTP/ATP, and reduced forms of NADH and FADH2 (Figure 5.28). This is considered an aerobic pathway because the NADH and FADH2 produced must transfer their electrons to the next pathway in the system, which will use oxygen. If this transfer does not occur, the oxidation steps of the citric acid cycle also do not occur. Note that the citric acid cycle produces very little ATP directly and does not directly consume oxygen.
Figure 5.28 In the citric acid cycle, the acetyl group from acetyl CoA is attached to a four-carbon oxaloacetate molecule to form a six-carbon citrate molecule. Through a series of steps, citrate is oxidized, releasing two carbon dioxide molecules for each acetyl group fed into the cycle. In the process, three NAD+ molecules are reduced to NADH, one FAD molecule is reduced to FADH2, and one ATP or GTP (depending on the cell type) is produced (by substrate-level phosphorylation). Because the final product of the citric acid cycle is also the first reactant, the cycle runs continuously in the presence of sufficient reactants. (credit: modification of work by “Yikrazuul”/Wikimedia Commons)
Click through each step of the citric acid cycle here (http://openstaxcollege.org/l/krebs_cycle) .
Products of the Citric Acid Cycle
Two carbon atoms come into the citric acid cycle from each acetyl group, representing four out of the six carbons of one glucose molecule. Two carbon dioxide molecules are released on each turn of the cycle; however, these do not necessarily contain the most recently added carbon atoms. The two acetyl carbon atoms will eventually be released on later turns of the cycle; thus, all six carbon atoms from the original glucose molecule are eventually incorporated into carbon dioxide. Each turn of the cycle forms three NADH molecules and one FADH2 molecule. These carriers will connect with the last portion of aerobic respiration to produce ATP molecules. One GTP or ATP is also made in each cycle. Several of the intermediate compounds in the citric acid cycle can be used in synthesizing non-essential amino acids; therefore, the cycle is amphibolic (both catabolic and anabolic).
5.7 Oxidative Phosphorylation
You have just read about two pathways in glucose catabolism—glycolysis and the citric acid cycle—that generate ATP. Most of the ATP generated during the aerobic catabolism of glucose, however, is not generated directly from these pathways. Rather, it is derived from a process that begins with moving electrons through a series of electron transporters that undergo redox reactions. This causes hydrogen ions to accumulate within the matrix space. Therefore, a concentration gradient forms in which hydrogen ions diffuse out of the matrix space by passing through ATP synthase. The current of hydrogen ions powers the catalytic action of ATP synthase, which phosphorylates ADP, producing ATP.
Electron Transport Chain
The electron transport chain (Figure 5.29) is the last component of aerobic respiration and is the only part of glucose metabolism that uses atmospheric oxygen. Oxygen continuously diffuses into plants; in animals, it enters the body through the respiratory system. Electron transport is a series of redox reactions that resemble a relay race or bucket brigade in that electrons are passed rapidly from one component to the next, to the endpoint of the chain where the electrons reduce molecular oxygen, producing water. There are four complexes composed of proteins, labeled I through IV in Figure 5.29, and the aggregation of these four complexes, together with associated mobile, accessory electron carriers, is called the electron transport chain. The electron transport chain is present in multiple copies in the inner mitochondrial membrane of eukaryotes and the plasma membrane of prokaryotes.
Figure 5.29 The electron transport chain is a series of electron transporters embedded in the inner mitochondrial membrane that shuttles electrons from NADH and FADH2 to molecular oxygen. In the process, protons are pumped from the mitochondrial matrix to the intermembrane space, and oxygen is reduced to form water.
Chemiosmosis
In chemiosmosis, the free energy from the series of redox reactions just described is used to pump hydrogen ions (protons) across the membrane. The uneven distribution of H+ ions across the membrane establishes both concentration and electrical gradients (thus, an electrochemical gradient), owing to the hydrogen ions’ positive charge and their aggregation on one side of the membrane.
If the membrane were open to diffusion by the hydrogen ions, the ions would tend to diffuse back across into the matrix, driven by their electrochemical gradient. Recall that many ions cannot diffuse through the nonpolar regions of phospholipid membranes without the aid of ion channels. Similarly, hydrogen ions in the matrix space can only pass through the inner mitochondrial membrane through an integral membrane protein called ATP synthase (Figure 5.30). This complex protein acts as a tiny generator, turned by the force of the hydrogen ions diffusing through it, down their electrochemical gradient. The turning of parts of this molecular machine facilitates the addition of a phosphate to ADP, forming ATP, using the potential energy of the hydrogen ion gradient.
Chemiosmosis (Figure 5.31) is used to generate 90 percent of the ATP made during aerobic glucose catabolism; it is also the method used in the light reactions of photosynthesis to harness the energy of sunlight in the process of photophosphorylation. Recall that the production of ATP using the process of chemiosmosis in mitochondria is called oxidative phosphorylation. The overall result of these reactions is the production of ATP from the energy of the electrons removed from hydrogen atoms. These atoms were originally part of a glucose molecule. At the end of the pathway, the electrons are used to reduce an oxygen molecule to oxygen ions. The extra electrons on the oxygen attract hydrogen ions (protons) from the surrounding medium, and water is formed.
ATP Yield
The number of ATP molecules generated from the catabolism of glucose varies. For example, the number of hydrogen ions that the electron transport chain complexes can pump through the membrane varies between species. Another source of variance stems from the shuttle of electrons across the membranes of the mitochondria. (The NADH generated from glycolysis cannot easily enter mitochondria.) Thus, electrons are picked up on the inside of mitochondria by either NAD+ or FAD+. As you have learned earlier, these FAD+ molecules can transport fewer ions; consequently, fewer ATP molecules are generated when FAD+ acts as a carrier. NAD+ is used as the electron transporter in the liver and FAD+ acts in the brain.
Another factor that affects the yield of ATP molecules generated from glucose is the fact that intermediate compounds in these pathways are used for other purposes. Glucose catabolism connects with the pathways that build or break down all other biochemical compounds in cells, and the result is somewhat messier than the ideal situations described thus far. For example, sugars other than glucose are fed into the glycolytic pathway for energy extraction. Moreover, the five-carbon sugars that form nucleic acids are made from intermediates in glycolysis. Certain nonessential amino acids can be made from intermediates of both glycolysis and the citric acid cycle. Lipids, such as cholesterol and triglycerides, are also made from intermediates in these pathways, and both amino acids and triglycerides are broken down for energy through these pathways. Overall, in living systems, these pathways of glucose catabolism extract about 34 percent of the energy contained in glucose.
5.8 | Metabolism without Oxygen
In aerobic respiration, the final electron acceptor is an oxygen molecule, O2. If aerobic respiration occurs, then ATP will be produced using the energy of high-energy electrons carried by NADH or FADH2 to the electron transport chain. If aerobic respiration does not occur, NADH must be reoxidized to NAD+ for reuse as an electron carrier for the glycolytic pathway to continue. How is this done? Some living systems use an organic molecule as the final electron acceptor. Processes that use an organic molecule to regenerate NAD+ from NADH are collectively referred to as fermentation. In contrast, some living systems use an inorganic molecule as a final electron acceptor. Both methods are called anaerobic cellular respiration in which organisms convert energy for their use in the absence of oxygen.
Anaerobic Cellular Respiration
Certain prokaryotes, including some species of bacteria and Archaea, use anaerobic respiration. For example, the group of Archaea called methanogens reduces carbon dioxide to methane to oxidize NADH. These microorganisms are found in soil and in the digestive tracts of ruminants, such as cows and sheep. Similarly, sulfate-reducing bacteria and Archaea, most of which are anaerobic ( Figure 5.32), reduce sulfate to hydrogen sulfide to regenerate NAD+ from NADH.
Figure 5.32 The green color seen in these coastal waters is from an eruption of hydrogen sulfide producing bacteria. These anaerobic, sulfate-reducing bacteria release hydrogen sulfide gas as they decompose algae in the water. (credit: modification of work by NASA/Jeff Schmaltz, MODIS Land Rapid Response Team at NASA GSFC, Visible Earth Catalog of NASA images)
Visit this site (http://openstaxcollege.org/l/fermentation) to see anaerobic cellular respiration in action.
Lactic Acid Fermentation
The fermentation method used by animals and certain bacteria, like those in yogurt, is lactic acid fermentation ( Figure 5.33). This type of fermentation is used routinely in mammalian red blood cells and in skeletal muscle that has an insufficient oxygen supply to allow aerobic respiration to continue (that is, in muscles used to the point of fatigue). In muscles, lactic acid accumulation must be removed by the blood circulation and the lactate brought to the liver for further metabolism. The chemical reactions of lactic acid fermentation are the following:
Pyruvic acid + NADH ↔ lactic acid + NAD+
The enzyme used in this reaction is lactate dehydrogenase (LDH). The reaction can proceed in either direction, but the reaction from left to right is inhibited by acidic conditions. Such lactic acid accumulation was once believed to cause muscle stiffness, fatigue, and soreness, although more recent research disputes this hypothesis. Once the lactic acid has been removed from the muscle and circulated to the liver, it can be reconverted into pyruvic acid and further catabolized for energy.
Alcohol Fermentation
Another familiar fermentation process is alcohol fermentation ( Figure 5.34) that produces ethanol, an alcohol. The first chemical reaction of alcohol fermentation is the following (CO2 does not participate in the second reaction):
Pyruvic acid → CO2 + acetaldehyde + NADH → ethanol + NAD+
The first reaction is catalyzed by pyruvate decarboxylase, a cytoplasmic enzyme, with a coenzyme of thiamine pyrophosphate (TPP, derived from vitamin B1 and also called thiamine). A carboxyl group is removed from pyruvic acid, releasing carbon dioxide as a gas. The loss of carbon dioxide reduces the size of the molecule by one carbon, making acetaldehyde. The second reaction is catalyzed by alcohol dehydrogenase to oxidize NADH to NAD+ and reduce acetaldehyde to ethanol. The fermentation of pyruvic acid by yeast produces the ethanol found in alcoholic beverages. Ethanol tolerance of yeast is variable, ranging from about 5 percent to 21 percent, depending on the yeast strain and environmental conditions.
Figure 5.34 Fermentation of grape juice into wine produces CO2 as a byproduct. Fermentation tanks have valves so that the pressure inside the tanks created by the carbon dioxide produced can be released.
Other Types of Fermentation
Other fermentation methods occur in bacteria. Many prokaryotes are facultatively anaerobic. This means that they can switch between aerobic respiration and fermentation, depending on the availability of oxygen. Certain prokaryotes, like Clostridia, are obligate anaerobes. Obligate anaerobes live and grow in the absence of molecular oxygen. Oxygen is a poison to these microorganisms and kills them on exposure. It should be noted that all forms of fermentation, except lactic acid fermentation, produce gas. The production of particular types of gas is used as an indicator of the fermentation of specific carbohydrates, which plays a role in the laboratory identification of the bacteria. Various methods of fermentation are used by assorted organisms to ensure an adequate supply of NAD+ for the sixth step in glycolysis. Without these pathways, that step would not occur and no ATP would be harvested from the breakdown of glucose.
5.9 | Connections of Carbohydrate, Protein, and Lipid Metabolic Pathways
You have learned about the catabolism of glucose, which provides energy to living cells. But living things consume more than glucose for food. How does a turkey sandwich end up as ATP in your cells? This happens because all of the catabolic pathways for carbohydrates, proteins, and lipids eventually connect into glycolysis and the citric acid cycle pathways (see Figure 5.36). Metabolic pathways should be thought of as porous—that is, substances enter from other pathways, and intermediates leave for other pathways. These pathways are not closed systems. Many of the substrates, intermediates, and products in a particular pathway are reactants in other pathways.
Connections of Other Sugars to Glucose Metabolism
Glycogen, a polymer of glucose, is an energy storage molecule in animals. When there is adequate ATP present, excess glucose is shunted into glycogen for storage. Glycogen is made and stored in both liver and muscle. The glycogen will be hydrolyzed into glucose monomers (G-1-P) if blood sugar levels drop. The presence of glycogen as a source of glucose allows ATP to be produced for a longer period of time during exercise. Glycogen is broken down into G-1-P and converted into G-6-P in both muscle and liver cells, and this product enters the glycolytic pathway.
Sucrose is a disaccharide with a molecule of glucose and a molecule of fructose bonded together with a glycosidic linkage. Fructose is one of the three dietary monosaccharides, along with glucose and galactose (which is part of the milk sugar, the disaccharide lactose), which are absorbed directly into the bloodstream during digestion. The catabolism of both fructose and galactose produces the same number of ATP molecules as glucose.
Connections of Proteins to Glucose Metabolism
Proteins are hydrolyzed by a variety of enzymes in cells. Most of the time, the amino acids are recycled into the synthesis of new proteins. If there are excess amino acids, however, or if the body is in a state of starvation, some amino acids will be shunted into the pathways of glucose catabolism (Figure 5.35). Each amino acid must have its amino group removed prior to entry into these pathways. The amino group is converted into ammonia. In mammals, the liver synthesizes urea from two ammonia molecules and a carbon dioxide molecule. Thus, urea is the principal waste product in mammals produced from the nitrogen originating in amino acids, and it leaves the body in urine.
Figure 5.35 The carbon skeletons of certain amino acids (indicated in boxes) derived from proteins can feed into the citric acid cycle. (credit: modification of work by Mikael Häggström)
Connections of Lipid and Glucose Metabolisms
The lipids that are connected to the glucose pathways are cholesterol and triglycerides. Cholesterol is a lipid that contributes to cell membrane flexibility and is a precursor of steroid hormones. The synthesis of cholesterol starts with acetyl groups and proceeds in only one direction. The process cannot be reversed.
Triglycerides are a form of long-term energy storage in animals. Triglycerides are made of glycerol and three fatty acids. Animals can make most of the fatty acids they need. Triglycerides can be both made and broken down through parts of the glucose catabolism pathways. Glycerol can be phosphorylated to glycerol-3-phosphate, which continues through glycolysis. Fatty acids are catabolized in a process called beta-oxidation that takes place in the matrix of the mitochondria and converts their fatty acid chains into two carbon units of acetyl groups. The acetyl groups are picked up by CoA to form acetyl CoA that proceeds into the citric acid cycle.
Figure 5.36 Glycogen from the liver and muscles, hydrolyzed into glucose-1-phosphate, together with fats and proteins, can feed into the catabolic pathways for carbohydrates.
5.10 | Regulation of Cellular Respiration
Cellular respiration must be regulated in order to provide balanced amounts of energy in the form of ATP. The cell also must generate a number of intermediate compounds that are used in the anabolism and catabolism of macromolecules. Without controls, metabolic reactions would quickly come to a stand still as the forward and backward reactions reached a state of equilibrium. Resources would be used inappropriately. A cell does not need the maximum amount of ATP that it can make all the time: At times, the cell needs to shunt some of the intermediates to pathways for amino acid, protein, glycogen, lipid, and nucleic acid production. In short, the cell needs to control its metabolism.
Regulatory Mechanisms
A variety of mechanisms is used to control cellular respiration. Some type of control exists at each stage of glucose metabolism. Access of glucose to the cell can be regulated using the GLUT proteins that transport glucose (Figure 5.37). Different forms of the GLUT protein control passage of glucose into the cells of specific tissues.
Figure 5.37 GLUT4 is a glucose transporter that is stored in vesicles. A cascade of events that occurs upon insulin binding to a receptor in the plasma membrane causes GLUT4-containing vesicles to fuse with the plasma membrane so that glucose may be transported into the cell.
Some reactions are controlled by having two different enzymes—one each for the two directions of a reversible reaction. Reactions that are catalyzed by only one enzyme can go to equilibrium, stalling the reaction. In contrast, if two different enzymes (each specific for a given direction) are necessary for a reversible reaction, the opportunity to control the rate of the reaction increases, and equilibrium is not reached.
A number of enzymes involved in each of the pathways—in particular, the enzyme catalyzing the first committed reaction of the pathway—are controlled by attachment of a molecule to an allosteric site on the protein. The molecules most commonly used in this capacity are the nucleotides ATP, ADP, AMP, NAD+, and NADH. These regulators, allosteric effectors, may increase or decrease enzyme activity, depending on the prevailing conditions. The allosteric effector alters the steric structure of the enzyme, usually affecting the configuration of the active site. This alteration of the protein’s (the enzyme’s) structure either increases or decreases its affinity for its substrate, with the effect of increasing or decreasing the rate of the reaction. The attachment signals to the enzyme. This binding can increase or decrease the enzyme’s activity, providing feedback. This feedback type of control is effective as long as the chemical affecting it is attached to the enzyme. Once the overall concentration of the chemical decreases, it will diffuse away from the protein, and the control is relaxed.
Control of Catabolic Pathways
Enzymes, proteins, electron carriers, and pumps that play roles in glycolysis, the citric acid cycle, and the electron transport chain tend to catalyze non-reversible reactions. In other words, if the initial reaction takes place, the pathway is committed to proceeding with the remaining reactions. Whether a particular enzyme activity is released depends upon the energy needs of the cell (as reflected by the levels of ATP, ADP, and AMP).
Electron Transport Chain
Specific enzymes of the electron transport chain are unaffected by feedback inhibition, but the rate of electron transport through the pathway is affected by the levels of ADP and ATP. Greater ATP consumption by a cell is indicated by a buildup of ADP. As ATP usage decreases, the concentration of ADP decreases, and now, ATP begins to build up in the cell. This change is the relative concentration of ADP to ATP triggers the cell to slow down the electron transport chain.
Visit this site (http://openstaxcollege.org/l/electron_transp) to see an animation of the electron transport chain and ATP synthesis.
5.11 | Overview of Photosynthesis
Photosynthesis is essential to all life on earth; both plants and animals depend on it. It is the only biological process that can capture energy that originates in outer space (sunlight) and convert it into chemical compounds (carbohydrates) that every organism uses to power its metabolism. In brief, the energy of sunlight is captured and used to energize electrons, which are then stored in the covalent bonds of sugar molecules. How long lasting and stable are those covalent bonds? The energy extracted today by the burning of coal and petroleum products represents sunlight energy captured and stored by photosynthesis almost 200 million years ago.
Plants, algae, and a group of bacteria called cyanobacteria are the only organisms capable of performing photosynthesis (Figure 5.38). Because they use light to manufacture their own food, they are called photoautotrophs (literally, “self-feeders using light”). Other organisms, such as animals, fungi, and most other bacteria, are termed heterotrophs (“other feeders”), because they must rely on the sugars produced by photosynthetic organisms for their energy needs. A third very interesting group of bacteria synthesize sugars, not by using sunlight’s energy, but by extracting energy from inorganic chemical compounds; hence, they are referred to as chemoautotrophs.
Figure 5.38 Photoautotrophs including (a) plants, (b) algae, and (c) cyanobacteria synthesize their organic compounds via photosynthesis using sunlight as an energy source. Cyanobacteria and planktonic algae can grow over enormous areas in water, at times completely covering the surface. In a (d) deep sea vent, chemoautotrophs, such as these (e) thermophilic bacteria, capture energy from inorganic compounds to produce organic compounds. The ecosystem surrounding the vents has a diverse array of animals, such as tubeworms, crustaceans, and octopi that derive energy from the bacteria. (credit a: modification of work by Steve Hillebrand, U.S. Fish and Wildlife Service; credit b: modification of work by “eutrophication&hypoxia”/Flickr; credit c: modification of work by NASA; credit d: University of Washington, NOAA; credit e: modification of work by Mark Amend, West Coast and Polar Regions Undersea Research Center, UAF, NOAA)
The importance of photosynthesis is not just that it can capture sunlight’s energy. A lizard sunning itself on a cold day can use the sun’s energy to warm up. Photosynthesis is vital because it evolved as a way to store the energy in solar radiation (the “photo-” part) as high-energy electrons in the carbon-carbon bonds of carbohydrate molecules (the “-synthesis” part). Those carbohydrates are the energy source that heterotrophs use to power the synthesis of ATP via respiration. Therefore, photosynthesis powers 99 percent of Earth’s ecosystems. When a top predator, such as a wolf, preys on a deer (Figure 5.39), the wolf is at the end of an energy path that went from nuclear reactions on the surface of the sun, to light, to photosynthesis, to vegetation, to deer, and finally to wolf.
Figure 5.39 The energy stored in carbohydrate molecules from photosynthesis passes through the food chain. The predator that eats these deer receives a portion of the energy that originated in the photosynthetic vegetation that the deer consumed. (credit: modification of work by Steve VanRiper,U.S. Fish and Wildlife Service)
Main Structures and Summary of Photosynthesis
Photosynthesis is a multi-step process that requires sunlight, carbon dioxide (which is low in energy), and water as substrates (Figure 5.40). After the process is complete, it releases oxygen and produces glyceraldehyde-3-phosphate (GA3P), simple carbohydrate molecules (which are high in energy) that can subsequently be converted into glucose, sucrose, or any of dozens of other sugar molecules. These sugar molecules contain energy and the energized carbon that all living things need to survive.
Figure 5.40 Photosynthesis uses solar energy, carbon dioxide, and water to produce energy-storing carbohydrates. Oxygen is generated as a waste product of photosynthesis.
The following is the chemical equation for photosynthesis (Figure 5.41):
Figure 5.41 The basic equation for photosynthesis is deceptively simple. In reality, the process takes place in many steps involving intermediate reactants and products. Glucose, the primary energy source in cells, is made from two three-carbon GA3Ps.
Although the equation looks simple, the many steps that take place during photosynthesis are actually quite complex. Before learning the details of how photoautotrophs turn sunlight into food, it is important to become familiar with the structures involved.In plants, photosynthesis generally takes place in leaves, which consist of several layers of cells. The process of photosynthesis occurs in a middle layer called the mesophyll. The gas exchange of carbon dioxide and oxygen occurs through small, regulated openings called stomata (singular: stoma), which also play roles in the regulation of gas exchange and water balance. The stomata are typically located on the underside of the leaf, which helps to minimize water loss. Each stoma is flanked by guard cells that regulate the opening and closing of the stomata by swelling or shrinking in response to osmotic changes.In all autotrophic eukaryotes, photosynthesis takes place inside an organelle called a chloroplast. For plants, chloroplast-containing cells exist in the mesophyll. Chloroplasts have a double membrane envelope (composed of an outer membrane and an inner membrane). Within the chloroplast are stacked, disc-shaped structures called thylakoids. Embedded in the thylakoid membrane is chlorophyll, a pigment (molecule that absorbs light) responsible for the initial interaction between light and plant material, and numerous proteins that make up the electron transport chain. The thylakoid membrane encloses an internal space called the thylakoid lumen. As shown in Figure 5.42, a stack of thylakoids is called a granum, and the liquid-filled space surrounding the granum is called stroma or “bed” (not to be confused with stoma or “mouth,” an opening on the leaf epidermis).
The Two Parts of Photosynthesis
Photosynthesis takes place in two sequential stages: the light-dependent reactions and the light independent-reactions. In the light-dependent reactions, energy from sunlight is absorbed by chlorophyll and that energy is converted into stored chemical energy. In the light-independent reactions, the chemical energy harvested during the light-dependent reactions drive the assembly of sugar molecules from carbon dioxide. Therefore, although the light-independent reactions do not use light as a reactant, they require the products of the light-dependent reactions to function. In addition, several enzymes of the light-independent reactions are activated by light. The light-dependent reactions utilize certain molecules to temporarily store the energy: These are referred to as energy carriers. The energy carriers that move energy from light-dependent reactions to light-independent reactions can be thought of as “full” because they are rich in energy. After the energy is released, the “empty” energy carriers return to the light-dependent reaction to obtain more energy. Figure 5.43 illustrates the components inside the chloroplast where the light-dependent and light-independent reactions take place.
Figure 5.43 Photosynthesis takes place in two stages: light dependent reactions and the Calvin cycle. Light-dependent reactions, which take place in the thylakoid membrane, use light energy to make ATP and NADPH. The Calvin cycle, which takes place in the stroma, uses energy derived from these compounds to make GA3P from CO2.Click the link (http://openstaxcollege.org/l/photosynthesis) to learn more about photosynthesis.
5.12 | The Light-Dependent Reactions of Photosynthesis
How can light be used to make food? When a person turns on a lamp, electrical energy becomes light energy. Like all other forms of kinetic energy, light can travel, change form, and be harnessed to do work. In the case of photosynthesis, light energy is converted into chemical energy, which photoautotrophs use to build carbohydrate molecules (Figure 5.44). However, autotrophs only use a few specific components of sunlight.
Figure 5.44 Photoautotrophs can capture light energy from the sun, converting it into the chemical energy used to build food molecules. (credit: Gerry Atwell)
What Is Light Energy?
The sun emits an enormous amount of electromagnetic radiation (solar energy). Humans can see only a fraction of this energy, which portion is therefore referred to as “visible light.” The manner in which solar energy travels is described as waves. Scientists can determine the amount of energy of a wave by measuring its wavelength, the distance between consecutive points of a wave. A single wave is measured from two consecutive points, such as from crest to crest or from trough to trough (Figure 5.45).
Figure 5.45 The wavelength of a single wave is the distance between two consecutive points of similar position (two crests or two troughs) along the wave.Visible light constitutes only one of many types of electromagnetic radiation emitted from the sun and other stars. Scientists differentiate the various types of radiant energy from the sun within the electromagnetic spectrum. The electromagnetic spectrum is the range of all possible frequencies of radiation (Figure 5.46). The difference between wavelengths relates to the amount of energy carried by them.
Figure 5.46 The sun emits energy in the form of electromagnetic radiation. This radiation exists at different wavelengths, each of which has its own characteristic energy. All electromagnetic radiation, including visible light, is characterized by its wavelength.
The electromagnetic spectrum (Figure 5.46) shows several types of electromagnetic radiation originating from the sun, including X-rays and ultraviolet (UV) rays. The higher-energy waves can penetrate tissues and damage cells and DNA, explaining why both X-rays and UV rays can be harmful to living organisms.
Absorption of Light
Light energy initiates the process of photosynthesis when pigments absorb the light. Organic pigments, whether in the human retina or the chloroplast thylakoid, have a narrow range of energy levels that they can absorb. Energy levels lower than those represented by red light are insufficient to raise an orbital electron to a populatable, excited (quantum) state. Energy levels higher than those in blue light will physically tear the molecules apart, called bleaching. So retinal pigments can only “see” (absorb) 700 nm to 400 nm light, which is therefore called visible light. For the same reasons, plants pigment molecules absorb only light in the wavelength range of 700 nm to 400 nm; plant physiologists refer to this range for plants as photosynthetically active radiation.
The visible light seen by humans as white light actually exists in a rainbow of colors. Certain objects, such as a prism or a drop of water, disperse white light to reveal the colors to the human eye. The visible light portion of the electromagnetic spectrum shows the rainbow of colors, with violet and blue having shorter wavelengths, and therefore higher energy. At the other end of the spectrum toward red, the wavelengths are longer and have lower energy (Figure 5.48).
Figure 5.48 The colors of visible light do not carry the same amount of energy. Violet has the shortest wavelength and therefore carries the most energy, whereas red has the longest wavelength and carries the least amount of energy. (credit: modification of work by NASA)
Understanding Pigments
Different kinds of pigments exist, and each has evolved to absorb only certain wavelengths (colors) of visible light. Pigments reflect or transmit the wavelengths they cannot absorb, making them appear in the corresponding color.
Each type of pigment can be identified by the specific pattern of wavelengths it absorbs from visible light, which is the absorption spectrum. The graph in Figure 5.48 shows the absorption spectra for chlorophyll a, chlorophyll b, and a type of carotenoid pigment called β-carotene (which absorbs blue and green light). Notice how each pigment has a distinct set of peaks and troughs, revealing a highly specific pattern of absorption. Chlorophyll a absorbs wavelengths from either end of the visible spectrum (blue and red), but not green. Because green is reflected or transmitted, chlorophyll appears green. Carotenoids absorb in the short-wavelength blue region, and reflect the longer yellow, red, and orange wavelengths.
Figure 5.49 (a) Chlorophyll a, (b) chlorophyll b, and (c) β-carotene are hydrophobic organic pigments found in the thylakoid membrane. Chlorophyll a and b, which are identical except for the part indicated in the red box, are responsible for the green color of leaves. β-carotene is responsible for the orange color in carrots. Each pigment has (d) a unique absorbance spectrum.
Many photosynthetic organisms have a mixture of pigments; using them, the organism can absorb energy from a wider range of wavelengths. Not all photosynthetic organisms have full access to sunlight. Some organisms grow underwater where light intensity and quality decrease and change with depth. Other organisms grow in competition for light. Plants on the rainforest floor must be able to absorb any bit of light that comes through, because the taller trees absorb most of the sunlight and scatter the remaining solar radiation (Figure 5.49).
Figure 5.49 Plants that commonly grow in the shade have adapted to low levels of light by changing the relative concentrations of their chlorophyll pigments. (credit: Jason Hollinger)
When studying a photosynthetic organism, scientists can determine the types of pigments present by generating absorption spectra. An instrument called a spectrophotometer can differentiate which wavelengths of light a substance can absorb. Spectrophotometers measure transmitted light and compute from it the absorption. By extracting pigments from leaves and placing these samples into a spectrophotometer, scientists can identify which wavelengths of light an organism can absorb. Additional methods for the identification of plant pigments include various types of chromatography that separate the pigments by their relative affinities to solid and mobile phases.
How Light-Dependent Reactions Work
The overall function of light-dependent reactions is to convert solar energy into chemical energy in the form of NADPH and ATP. This chemical energy supports the light-independent reactions and fuels the assembly of sugar molecules. The light-dependent reactions are depicted in Figure 5.50. Protein complexes and pigment molecules work together to produce NADPH and ATP.
Figure 5.50 A photosystem consists of a light-harvesting complex and a reaction center. Pigments in the light-harvesting complex pass light energy to two special chlorophyll a molecules in the reaction center. The light excites an electron from the chlorophyll a pair, which passes to the primary electron acceptor. The excited electron must then be replaced. In (a) photosystem II, the electron comes from the splitting of water, which releases oxygen as a waste product. In (b) photosystem I, the electron comes from the chloroplast electron transport chain discussed below.
Both photosystems have the same basic structure; a number of antenna proteins to which the chlorophyll molecules are bound surround the reaction center where the photochemistry takes place. Each photosystem is serviced by the light-harvesting complex, which passes energy from sunlight to the reaction center; it consists of multiple antenna proteins that contain a mixture of 300–400 chlorophyll a and b molecules as well as other pigments like carotenoids. The absorption of a single photon or distinct quantity or “packet” of light by any of the chlorophylls pushes that molecule into an excited state. In short, the light energy has now been captured by biological molecules but is not stored in any useful form yet. The energy is transferred from chlorophyll to chlorophyll until eventually (after about a millionth of a second), it is delivered to the reaction center. Up to this point, only energy has been transferred between molecules, not electrons.
Generating an Energy Carrier: ATP
As in the intermembrane space of the mitochondria during cellular respiration, the buildup of hydrogen ions inside the thylakoid lumen creates a concentration gradient. The passive diffusion of hydrogen ions from high concentration (in the thylakoid lumen) to low concentration (in the stroma) is harnessed to create ATP, just as in the electron transport chain of cellular respiration. The ions build up energy because of diffusion and because they all have the same electrical charge, repelling each other.
To release this energy, hydrogen ions will rush through any opening, similar to water jetting through a hole in a dam. In the thylakoid, that opening is a passage through a specialized protein channel called the ATP synthase. The energy released by the hydrogen ion stream allows ATP synthase to attach a third phosphate group to ADP, which forms a molecule of ATP (Figure 5.50). The flow of hydrogen ions through ATP synthase is called chemiosmosis because the ions move from an area of high to an area of low concentration through a semi-permeable structure.
Visit this site (http://openstaxcollege.org/l/light_reactions) and click through the animation to view the process of photosynthesis within a leaf.
5.13 | Using Light Energy to Make Organic Molecules
After the energy from the sun is converted into chemical energy and temporarily stored in ATP and NADPH molecules, the cell has the fuel needed to build carbohydrate molecules for long-term energy storage. The products of the light-dependent reactions, ATP and NADPH, have lifespans in the range of millionths of seconds, whereas the products of the light-independent reactions (carbohydrates and other forms of reduced carbon) can survive for hundreds of millions of years. The carbohydrate molecules made will have a backbone of carbon atoms. Where does the carbon come from? It comes from carbon dioxide, the gas that is a waste product of respiration in microbes, fungi, plants, and animals.
The Calvin Cycle
In plants, carbon dioxide (CO2) enters the leaves through stomata, where it diffuses over short distances through intercellular spaces until it reaches the mesophyll cells. Once in the mesophyll cells, CO2 diffuses into the stroma of the chloroplast—the site of light-independent reactions of photosynthesis. These reactions actually have several names associated with them. Another term, the Calvin cycle, is named for the man who discovered it, and because these reactions function as a cycle.
Figure 5.51 Light reactions harness energy from the sun to produce chemical bonds, ATP, and NADPH. These energy-carrying molecules are made in the stroma where carbon fixation takes place.
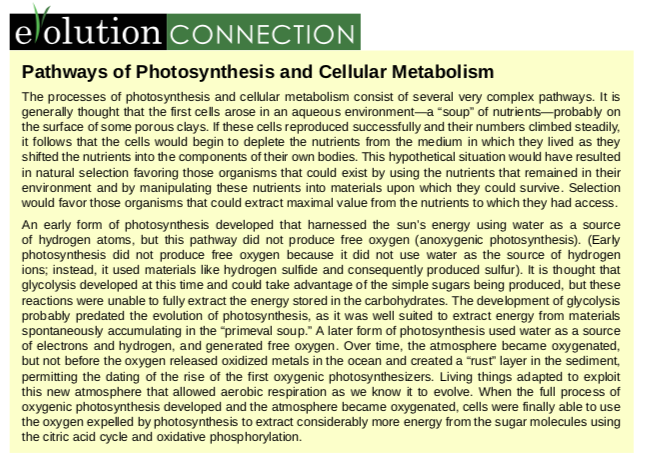
Photosynthesis During the evolution of photosynthesis, a major shift occurred from the bacterial type of photosynthesis that involves only one photosystem and is typically anoxygenic (does not generate oxygen) into modern oxygenic (does generate oxygen) photosynthesis, employing two photosystems. This modern oxygenic photosynthesis is used by many organisms—from giant tropical leaves in the rainforest to tiny cyanobacterial cells—and the process and components of this photosynthesis remain largely the same. Photosystems absorb light and use electron transport chains to convert energy into the chemical energy of ATP and NADH. The subsequent light-independent reactions then assemble carbohydrate molecules with this energy.
The Energy Cycle
Whether the organism is a bacterium, plant, or animal, all living things access energy by breaking down carbohydrate molecules. But if plants make carbohydrate molecules, why would they need to break them down, especially when it has been shown that the gas organisms release as a “waste product” (CO2) acts as a substrate for the formation of more food in photosynthesis? Remember, living things need energy to perform life functions. In addition, an organism can either make its own food or eat another organism—either way, the food still needs to be broken down. Finally, in the process of breaking down food, called cellular respiration, heterotrophs release needed energy and produce “waste” in the form of CO2 gas.
In nature, there is no such thing as waste. Every single atom of matter and energy is conserved, recycling over and over infinitely. Substances change form or move from one type of molecule to another, but their constituent atoms never disappear (Figure 5.53).
CO2 is no more a form of waste than oxygen is wasteful to photosynthesis. Both are byproducts of reactions that move on to other reactions. Photosynthesis absorbs light energy to build carbohydrates in chloroplasts, and aerobic cellular respiration releases energy by using oxygen to metabolize carbohydrates in the cytoplasm and mitochondria. Both processes use electron transport chains to capture the energy necessary to drive other reactions. These two powerhouse processes, photosynthesis and cellular respiration, function in biological, cyclical harmony to allow organisms to access life-sustaining energy that originates millions of miles away in a burning star humans call the sun.
Figure 5.54 Photosynthesis consumes carbon dioxide and produces oxygen. Aerobic respiration consumes oxygen and produces carbon dioxide. These two processes play an important role in the carbon cycle. (credit: modification of work by Stuart Bassil)
KEY TERMS
absorption spectrum range of wavelengths of electromagnetic radiation absorbed by a given substance
antenna protein pigment molecule that directly absorbs light and transfers the energy absorbed to other pigment molecules
Calvin cycle light-independent reactions of photosynthesis that convert carbon dioxide from the atmosphere into carbohydrates using the energy and reducing power of ATP and NADPH
carbon fixation process of converting inorganic CO2 gas into organic compounds
carotenoid photosynthetic pigment that functions to dispose of excess energy
chemoautotroph organism that can build organic molecules using energy derived from inorganic chemicals instead of sunlight
chlorophyll a form of chlorophyll that absorbs violet-blue and red light and consequently has a bluish-green color; the only pigment molecule that performs the photochemistry by getting excited and losing an electron to the electron transport chain
chlorophyll b accessory pigment that absorbs blue and red-orange light and consequently has a yellowish-green tint
chloroplast organelle in which photosynthesis takes place
cytochrome complex group of reversibly oxidizable and reducible proteins that forms part of the electron transport chain between photosystem II and photosystem I
electromagnetic spectrum range of all possible frequencies of radiation
electron transport chain group of proteins between PSII and PSI that pass energized electrons and use the energy released by the electrons to move hydrogen ions against their concentration gradient into the thylakoid lumen
granum stack of thylakoids located inside a chloroplast
heterotroph organism that consumes organic substances or other organisms for food
light harvesting complex complex that passes energy from sunlight to the reaction center in each photosystem; it consists of multiple antenna proteins that contain a mixture of 300–400 chlorophyll a and b molecules as well as other pigments like carotenoids
light-dependent reaction first stage of photosynthesis where certain wavelengths of the visible light are absorbed to form two energy-carrying molecules (ATP and NADPH)
light-independent reaction second stage of photosynthesis, though which carbon dioxide is used to build carbohydrate molecules using energy from ATP and NADPH
mesophyll middle layer of chlorophyll-rich cells in a leaf
photoautotroph organism capable of producing its own organic compounds from sunlight
photon distinct quantity or “packet” of light energy
photosystem group of proteins, chlorophyll, and other pigments that are used in the light dependent reactions of photosynthesis to absorb light energy and convert it into chemical energy
photosystem I integral pigment and protein complex in thylakoid membranes that uses light energy to transport electrons from plastocyanin to NADP+ (which becomes reduced to NADPH in the process)
photosystem II integral protein and pigment complex in thylakoid membranes that transports electrons from water to the electron transport chain; oxygen is a product of PSII
pigment molecule that is capable of absorbing certain wavelengths of light and reflecting others (which accounts for its color)
primary electron acceptor pigment or other organic molecule in the reaction center that accepts an energized electron from the reaction center
reaction center complex of chlorophyll molecules and other organic molecules that is assembled around a special pair of chlorophyll molecules and a primary electron acceptor; capable of undergoing oxidation and reduction
reduction gain of electron(s) by an atom or molecule
spectrophotometer instrument that can measure transmitted light and compute the absorption
stoma opening that regulates gas exchange and water evaporation between leaves and the environment, typically situated on the underside of leaves
stroma fluid-filled space surrounding the grana inside a chloroplast where the light-independent reactions of photosynthesis take place
thylakoid disc-shaped, membrane-bound structure inside a chloroplast where the light-dependent reactions of photosynthesis take place; stacks of thylakoids are called grana
thylakoid lumen aqueous space bound by a thylakoid membrane where protons accumulate during light-driven electron transport
wavelength distance between consecutive points of equal position (two crests or two troughs) of a wave in a graphic representation; inversely proportional to the energy of the radiation
activation energy energy necessary for reactions to occur
active site specific region of the enzyme to which the substrate binds
allosteric inhibition inhibition by a binding event at a site different from the active site, which induces a conformational change and reduces the affinity of the enzyme for its substrate
anabolic (also, anabolism) pathways that require an input of energy to synthesize complex molecules from simpler ones
ATP adenosine triphosphate, the cell’s energy currency
bioenergetics study of energy flowing through living systems
catabolic (also, catabolism) pathways in which complex molecules are broken down into simpler ones chemical energy potential energy in chemical bonds that is released when those bonds are broken
coenzyme small organic molecule, such as a vitamin or its derivative, which is required to enhance the activity of an enzyme
cofactor inorganic ion, such as iron and magnesium ions, required for optimal regulation of enzyme activity
competitive inhibition type of inhibition in which the inhibitor competes with the substrate molecule by binding to the active site of the enzyme
denature process that changes the natural properties of a substance
endergonic describes chemical reactions that require energy input
enthalpy total energy of a system
feedback inhibition effect of a product of a reaction sequence to decrease its further production by inhibiting the activity of the first enzyme in the pathway that produces it
induced fit dynamic fit between the enzyme and its substrate, in which both components modify their structures to allow for ideal binding
metabolism all the chemical reactions that take place inside cells, including anabolism and catabolism
substrate molecule on which the enzyme acts
transition state high-energy, unstable state (an intermediate form between the substrate and the product) occurring during a chemical reaction
acetyl CoA combination of an acetyl group derived from pyruvic acid and coenzyme A, which is made from pantothenic acid (a B-group vitamin)
aerobic respiration process in which organisms convert energy in the presence of oxygen
anaerobic process that does not use oxygen
anaerobic cellular respiration process in which organisms convert energy for their use in the absence of oxygen
ATP synthase (also, F1F0 ATP synthase) membrane-embedded protein complex that adds a phosphate to ADP with energy from protons diffusing through it
chemiosmosis process in which there is a production of adenosine triphosphate (ATP) in cellular metabolism by the involvement of a proton gradient across a membrane
citric acid cycle (also, Krebs cycle) series of enzyme-catalyzed chemical reactions of central importance in all living cells
dephosphorylation removal of a phosphate group from a molecule
fermentation process of regenerating NAD+ with either an inorganic or organic compound serving as the final electron acceptor, occurs in the absence; occurs in the absence of oxygen
GLUT protein integral membrane protein that transports glucose
glycolysis process of breaking glucose into two three-carbon molecules with the production of ATP and NADH
isomerase enzyme that converts a molecule into its isomer
Krebs cycle (also, citric acid cycle) alternate name for the citric acid cycle, named after Hans Krebs who first identified the steps in the pathway in the 1930s in pigeon flight muscles; see citric acid cycle
oxidative phosphorylation production of ATP using the process of chemiosmosis and oxygen
phosphorylation addition of a high-energy phosphate to a compound, usually a metabolic intermediate, a protein, or ADP
prosthetic group (also, prosthetic cofactor) molecule bound to a protein that facilitates the function of the protein
pyruvate three-carbon sugar that can be decarboxylated and oxidized to make acetyl CoA, which enters the citric acid cycle under aerobic conditions; the end product of glycolysis
redox reaction chemical reaction that consists of the coupling of an oxidation reaction and a reduction reaction
substrate-level phosphorylation production of ATP from ADP using the excess energy from a chemical reaction and a phosphate group from a reactant
TCA cycle (also, citric acid cycle) alternate name for the citric acid cycle, named after the group name for citric acid, tricarboxylic acid (TCA); see citric acid cycle
ubiquinone soluble electron transporter in the electron transport chain that connects the first or second complex to the third
CHAPTER SUMMARY
6.1 Energy and Metabolism
Cells perform the functions of life through various chemical reactions. A cell’s metabolism refers to the chemical reactions that take place within it. There are metabolic reactions that involve the breaking down of complex chemicals into simpler ones, such as the breakdown of large macromolecules. This process is referred to as catabolism, and such reactions are associated with a release of energy. On the other end of the spectrum, anabolism refers to metabolic processes that build complex molecules out of simpler ones, such as the synthesis of macromolecules. Anabolic processes require energy. Glucose synthesis and glucose breakdown are examples of anabolic and catabolic pathways, respectively.
6.2 ATP: Adenosine Triphosphate
ATP is the primary energy-supplying molecule for living cells. ATP is made up of a nucleotide, a five carbon sugar, and three phosphate groups. The bonds that connect the phosphates (phosphoanhydride bonds) have high-energy content. The energy released from the hydrolysis of ATP into ADP + Pi is used to perform cellular work. Cells use ATP to perform work by coupling the exergonic reaction of ATP hydrolysis with endergonic reactions. ATP donates its phosphate group to another molecule via a process known as phosphorylation. The phosphorylated molecule is at a higher-energy state and is less stable than its unphosphorylated form, and this added energy from the addition of the phosphate allows the molecule to undergo its endergonic reaction.
6.3 Enzymes
Enzymes are chemical catalysts that accelerate chemical reactions at physiological temperatures by lowering their activation energy. Enzymes are usually proteins consisting of one or more polypeptide chains. Enzymes have an active site that provides a unique chemical environment, made up of certain amino acid R groups (residues). This unique environment is perfectly suited to convert particular chemical reactants for that enzyme, called substrates, into unstable intermediates called transition states. Enzymes and substrates are thought to bind with an induced fit, which means that enzymes undergo slight conformational adjustments upon substrate contact, leading to full, optimal binding. Enzymes bind to substrates and catalyze reactions in four different ways: bringing substrates together in an optimal orientation, compromising the bond structures of substrates so that bonds can be more easily broken, providing optimal environmental conditions for a reaction to occur, or participating directly in their chemical reaction by forming transient covalent bonds with the substrates.
Enzyme action must be regulated so that in a given cell at a given time, the desired reactions are being catalyzed and the undesired reactions are not. Enzymes are regulated by cellular conditions, such as temperature and pH. They are also regulated through their location within a cell, sometimes being compartmentalized so that they can only catalyze reactions under certain circumstances. Inhibition and activation of enzymes via other molecules are other important ways that enzymes are regulated. Inhibitors can act competitively, noncompetitively, or allosterically; noncompetitive inhibitors are usually allosteric. Activators can also enhance the function of enzymes allosterically. The most common method by which cells regulate the enzymes in metabolic pathways is through feedback inhibition. During feedback inhibition, the products of a metabolic pathway serve as inhibitors (usually allosteric) of one or more of the enzymes (usually the first committed enzyme of the pathway) involved in the pathway that produces them.
654 Energy in Living Systems
ATP functions as the energy currency for cells. It allows the cell to store energy briefly and transport it within the cell to support endergonic chemical reactions. The structure of ATP is that of an RNA nucleotide with three phosphates attached. As ATP is used for energy, a phosphate group or two are detached, and either ADP or AMP is produced. Energy derived from glucose catabolism is used to convert ADP into ATP. When ATP is used in a reaction, the third phosphate is temporarily attached to a substrate in a process called phosphorylation. The two processes of ATP regeneration that are used in conjunction with glucose catabolism are substrate-level phosphorylation and oxidative phosphorylation through the process of chemiosmosis.
5.5 Glycolysis
Glycolysis is the first pathway used in the breakdown of glucose to extract energy. It was probably one of the earliest metabolic pathways to evolve and is used by nearly all of the organisms on earth. Glycolysis consists of two parts: The first part prepares the six-carbon ring of glucose for cleavage into two three-carbon sugars. ATP is invested in the process during this half to energize the separation. The second half of glycolysis extracts ATP and high-energy electrons from hydrogen atoms and attaches them to NAD+. Two ATP molecules are invested in the first half and four ATP molecules are formed by substrate phosphorylation during the second half. This produces a net gain of two ATP and two NADH molecules for the cell.
5.6 Oxidation of Pyruvate and the Citric Acid Cycle
In the presence of oxygen, pyruvate is transformed into an acetyl group attached to a carrier molecule of coenzyme A. The resulting acetyl CoA can enter several pathways, but most often, the acetyl group is delivered to the citric acid cycle for further catabolism. During the conversion of pyruvate into the acetyl group, a molecule of carbon dioxide and two high-energy electrons are removed. The carbon dioxide accounts for two (conversion of two pyruvate molecules) of the six carbons of the original glucose molecule. The electrons are picked up by NAD+, and the NADH carries the electrons to a later pathway for ATP production. At this point, the glucose molecule that originally entered cellular respiration has been completely oxidized. Chemical potential energy stored within the glucose molecule has been transferred to electron carriers or has been used to synthesize a few ATPs.
The citric acid cycle is a series of redox and decarboxylation reactions that remove high-energy electrons and carbon dioxide. The electrons temporarily stored in molecules of NADH and FADH2 are used to generate ATP in a subsequent pathway. One molecule of either GTP or ATP is produced by substrate-level phosphorylation on each turn of the cycle. There is no comparison of the cyclic pathway with a linear one.
5.7 Oxidative Phosphorylation
The electron transport chain is the portion of aerobic respiration that uses free oxygen as the final electron acceptor of the electrons removed from the intermediate compounds in glucose catabolism. The electron transport chain is composed of four large, multiprotein complexes embedded in the inner mitochondrial membrane and two small diffusible electron carriers shuttling electrons between them. The electrons are passed through a series of redox reactions, with a small amount of free energy used at three points to transport hydrogen ions across a membrane. This process contributes to the gradient used in chemiosmosis. The electrons passing through the electron transport chain gradually lose energy, High-energy electrons donated to the chain by either NADH or FADH2 complete the chain, as low energy electrons reduce oxygen molecules and form water. The level of free energy of the electrons drops from about 60 kcal/mol in NADH or 45 kcal/mol in FADH2 to about 0 kcal/mol in water. The end products of the electron transport chain are water and ATP. A number of intermediate compounds of the citric acid cycle can be diverted into the anabolism of other biochemical molecules, such as nonessential amino acids, sugars, and lipids. These same molecules can serve as energy sources for the glucose pathways.
5.8 Metabolism without Oxygen
If NADH cannot be oxidized through aerobic respiration, another electron acceptor is used. Most organisms will use some form of fermentation to accomplish the regeneration of NAD+, ensuring the continuation of glycolysis. The regeneration of NAD+ in fermentation is not accompanied by ATP production; therefore, the potential of NADH to produce ATP using an electron transport chain is not utilized.
5.9 Connections of Carbohydrate, Protein, and Lipid Metabolic Pathways
The breakdown and synthesis of carbohydrates, proteins, and lipids connect with the pathways of glucose catabolism. The simple sugars are galactose, fructose, glycogen, and pentose. These are catabolized during glycolysis. The amino acids from proteins connect with glucose catabolism through pyruvate, acetyl CoA, and components of the citric acid cycle. Cholesterol synthesis starts with acetyl groups, and the components of triglycerides come from glycerol-3-phosphate from glycolysis and acetyl groups produced in the mitochondria from pyruvate.
5.10 Regulation of Cellular Respiration
Cellular respiration is controlled by a variety of means. The entry of glucose into a cell is controlled by the transport proteins that aid glucose passage through the cell membrane. Most of the control of the respiration processes is accomplished through the control of specific enzymes in the pathways. This is a type of negative feedback, turning the enzymes off. The enzymes respond most often to the levels of the available nucleosides ATP, ADP, AMP, NAD+, and FAD. Other intermediates of the pathway also affect certain enzymes in the systems.
5.11 Overview of Photosynthesis
The process of photosynthesis transformed life on Earth. By harnessing energy from the sun, photosynthesis evolved to allow living things access to enormous amounts of energy. Because of photosynthesis, living things gained access to sufficient energy that allowed them to build new structures and achieve the biodiversity evident today.
Only certain organisms, called photoautotrophs, can perform photosynthesis; they require the presence of chlorophyll, a specialized pigment that absorbs certain portions of the visible spectrum and can capture energy from sunlight. Photosynthesis uses carbon dioxide and water to assemble carbohydrate molecules and release oxygen as a waste product into the atmosphere. Eukaryotic autotrophs, such as plants and algae, have organelles called chloroplasts in which photosynthesis takes place, and starch accumulates. In prokaryotes, such as cyanobacteria, the process is less localized and occurs within folded membranes, extensions of the plasma membrane, and in the cytoplasm.
5.12 The Light-Dependent Reactions of Photosynthesis
The pigments of the first part of photosynthesis, the light-dependent reactions, absorb energy from sunlight. A photon strikes the antenna pigments of photosystem II to initiate photosynthesis. The energy travels to the reaction center that contains chlorophyll a to the electron transport chain, which pumps hydrogen ions into the thylakoid interior. This action builds up a high concentration of ions. The ions flow through ATP synthase via chemiosmosis to form molecules of ATP, which are used for the formation of sugar molecules in the second stage of photosynthesis. Photosystem I absorbs a second photon, which results in the formation of an NADPH molecule, another energy and reducing power carrier for the light-independent reactions.
5.13 Using Light Energy to Make Organic Molecules
Using the energy carriers formed in the first steps of photosynthesis, the light-independent reactions, or the Calvin cycle, take in CO2 from the environment. An enzyme, RuBisCO, catalyzes a reaction with CO2 and another molecule, RuBP. After three cycles, a three-carbon molecule of G3P leaves the cycle to become part of a carbohydrate molecule. The remaining G3P molecules stay in the cycle to be regenerated into RuBP, which is then ready to react with more CO2. Photosynthesis forms an energy cycle with the process of cellular respiration. Plants need both photosynthesis and respiration for their ability to function in both the light and dark, and to be able to interconvert essential metabolites. Therefore, plants contain both chloroplasts and mitochondria.
REVIEW QUESTIONS
Energy is stored long-term in the bonds of _____ and used short-term to perform work from a(n) _____ molecule.
ATP : glucose
an anabolic molecule : catabolic molecule
glucose : ATP
a catabolic molecule : anabolic molecule
DNA replication involves unwinding two strands of parent DNA, copying each strand to synthesize complementary strands, and releasing the parent and daughter DNA. Which of the following accurately describes this process?
This is an anabolic process
This is a catabolic process
This is both anabolic and catabolic
This is a metabolic process but is neither anabolic nor catabolic
Which of the following is not an example of an energy transformation?
Turning on a light switch
Solar panels at work
Formation of static electricity
None of the above
The energy released by the hydrolysis of ATP is
primarily stored between the alpha and beta phosphates
equal to −57 kcal/mol
harnessed as heat energy by the cell to perform work
providing energy to coupled reactions
An allosteric inhibitor does which of the following?
Binds to an enzyme away from the active site and changes the conformation of the active site,
increasing its affinity for substrate binding
Binds to the active site and blocks it from binding substrate
Binds to an enzyme away from the active site and changes the conformation of the active site, decreasing its affinity for the substrate
Binds directly to the active site and mimics the substrate
Which of the following analogies best describe the induced-fit model of enzyme substrate binding?
A hug between two people
A key fitting into a lock
A square peg fitting through the square hole and a round peg fitting through the round hole of a children’s toy
The fitting together of two jigsaw puzzle pieces.
The energy currency used by cells is ________.
ATP
ADP
AMP
adenosine
Chemiosmosis involves ________.
the movement of electrons across the cell membrane
the movement of hydrogen atoms across a mitochondrial membrane
the movement of hydrogen ions across a mitochondrial membrane
the movement of glucose through the cell membrane
Which of the following fermentation methods can occur in animal skeletal muscles?
lactic acid fermentation
alcohol fermentation
mixed acid fermentation
propionic fermentation
The effect of high levels of ADP is to ________.
increase the activity of the enzyme
decrease the activity of the enzyme
have no effect on the activity of the enzyme
slow down the pathway
Which of the following components is not used by both plants and cyanobacteria to carry out photosynthesis?
chloroplasts
chlorophyll
carbon dioxide
water
What two main products result from photosynthesis?
oxygen and carbon dioxide
chlorophyll and oxygen
sugars/carbohydrates and oxygen
sugars/carbohydrates and carbon dioxide
CRITICAL THINKING QUESTIONS
Does physical exercise involve anabolic and/ or catabolic processes? Give evidence for your answer.
Name two different cellular functions that require energy that parallel human energy requiring functions.
Imagine an elaborate ant farm with tunnels and passageways through the sand where ants live in a large community. Now imagine that an earthquake shook the ground and demolished the ant farm. In which of these two scenarios, before or after the earthquake, was the ant farm system in a state of higher or lower entropy?
With regard to enzymes, why are vitamins necessary for good health? Give examples.
Why is it beneficial for cells to use ATP rather than energy directly from the bonds of carbohydrates? What are the greatest drawbacks to harnessing energy directly from the bonds of several different compounds?
Nearly all organisms on earth carry out some form of glycolysis. How does that fact support or not support the assertion that glycolysis is one of the oldest metabolic pathways?
Red blood cells do not perform aerobic respiration, but they do perform glycolysis. Why do all cells need an energy source, and what would happen if glycolysis were blocked in a red blood cell?
What is the primary difference between fermentation and anaerobic respiration?
Why might negative feedback mechanisms be more common than positive feedback mechanisms in living cells?
What is the overall outcome of the light reactions in photosynthesis?
Why are carnivores, such as lions, dependent on photosynthesis to survive?
Adapted from:
OpenStax, Biology. OpenStax. May 20, 2013. <http://cnx.org/content/col11448/latest/>
“Download for free at http://cnx.org/content/col11448/latest/.”