14 Background: DNA and the Polymerase Chain Reaction
DNA Structure
Nucleotides
DNA and RNA are comprised of monomers called nucleotides. The nucleotides combine with each other to form a polynucleotide, DNA or RNA. Three components comprise each nucleotide: a nitrogenous base, a pentose (five-carbon) sugar, and one or more phosphate groups. Each nitrogenous base in a nucleotide is attached to a sugar molecule, which is attached to one or more phosphate groups.
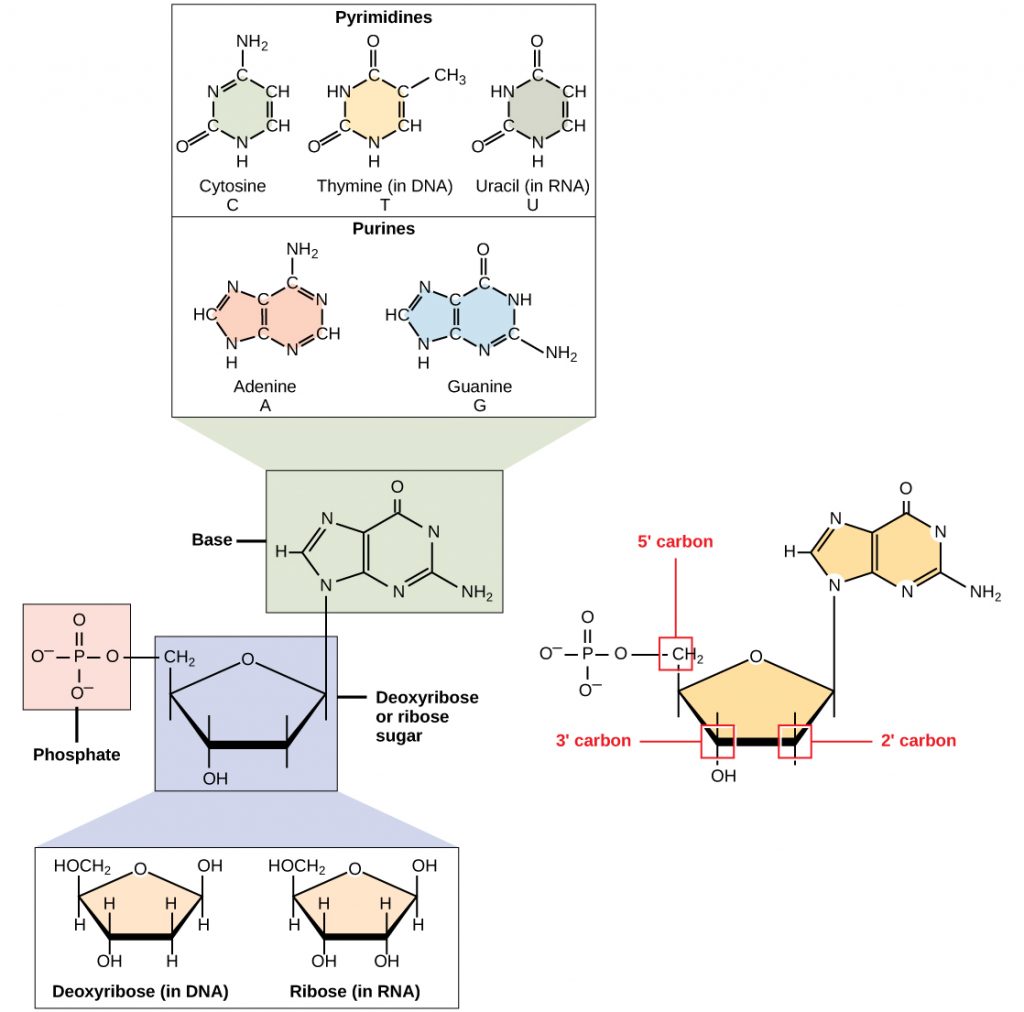
The nitrogenous bases are organic molecules that contain nitrogen. They are bases because they contain an amino group that has the potential of binding an extra hydrogen, and thus decreasing the hydrogen ion concentration in its environment, making it more basic. Each nucleotide in DNA contains one of four possible nitrogenous bases: adenine (A), guanine (G) cytosine (C), and thymine (T).
Scientists classify adenine and guanine as purines. The purine’s primary structure is two carbon-nitrogen rings. Scientists classify cytosine, thymine, and uracil as pyrimidines which have a single carbon-nitrogen ring as their primary structure. Each of these basic carbon-nitrogen rings has different functional groups attached to it. In molecular biology shorthand, we use the symbols A, T, G, C, and U for the nitrogenous bases. DNA contains A, T, G, and C; whereas, RNA contains A, U, G, and C.
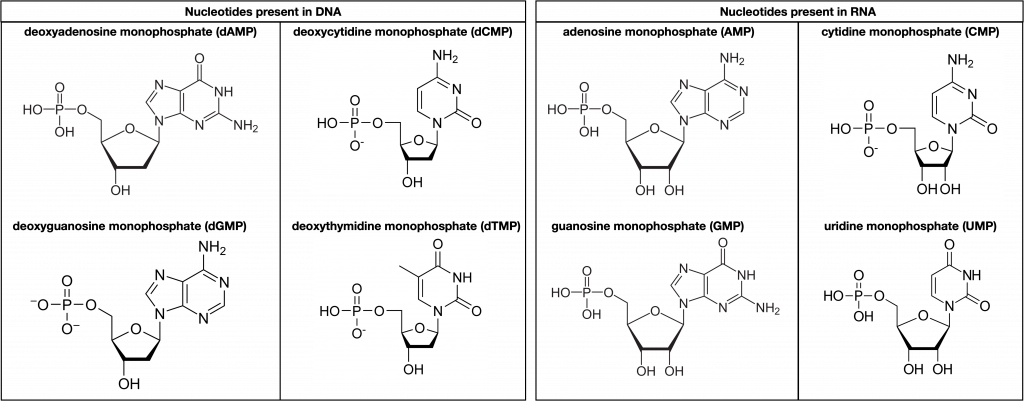
The pentose sugar in DNA is deoxyribose, and in RNA, the sugar is ribose. The difference between the sugars is the presence of the hydroxyl group on the ribose’s second carbon and hydrogen on the deoxyribose’s second carbon. The carbon atoms of the sugar molecule are numbered as 1′, 2′, 3′, 4′, and 5′ (1′ is read as “one prime”).
Polynucleotides
A phosphate residue connects the 5′ carbon of one sugar to the 3′ carbon of the sugar of the next nucleotide, which forms a 5′–3′ phosphodiester linkage. A polynucleotide may have thousands or even millions of phosphodiester linkages.
In a polynucleotide, one end of the chain has a free 5′ phosphate, and the other end has a free 3′-OH. These are called the 5′ and 3′ ends of the chain.
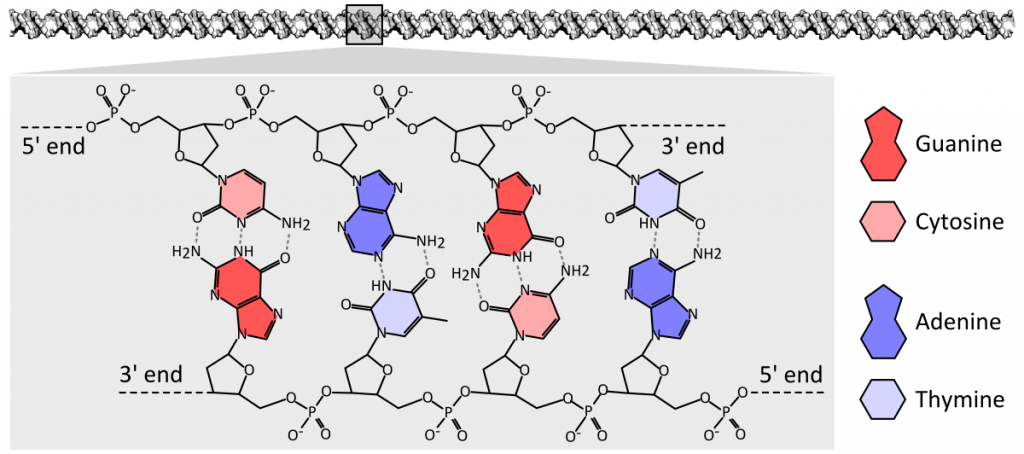
The Double Helix
DNA has a double-helix structure. The sugar and phosphate lie on the outside of the helix, forming the DNA’s backbone. The nitrogenous bases are stacked in the interior, like a pair of staircase steps. Hydrogen bonds bind the pairs to each other. Every base pair in the double helix is separated from the next base pair by 0.34 nm. There are about 10 base pairs per turn of the double helix. The helix’s two strands are antiparallel, meaning that they run in opposite directions.
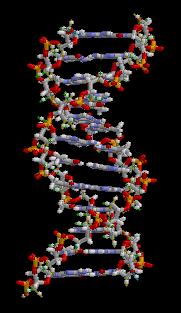
Base pairing is specific. A can pair with T, and G can pair with C. This is the base complementary rule. In other words, the DNA strands are complementary to each other. If the sequence of one strand is 5′-AATTGGCC-3′, the complementary strand would have the sequence 3′-TTAACCGG-5′.
The Polymerase Chain Reaction
The polymerase chain reaction laboratory technique is used in a variety of applications to make copies of a specific DNA sequence. This lesson describes how a PCR reaction works, what it accomplishes, and its basic requirements for success. Examples of interpreting results are given. PCR’s strengths, weaknesses, and applications to plant biotechnology are explained.
PCR is an In Vitro process; a series of chemical reactions that happen outside of a living cell. This laboratory technique is modeled after an In vivo process, the living cell’s natural ability to replicate DNA during normal cell cycles. Every living cell makes a duplicate copy of each chromosome before the cell is ready to divide. Figure 1 below illustrates the key parts of In vivo DNA replication that are the basis for PCR success.
PCR works as an in vitro DNA replication process by using just one of these enzymes.
Name and Chemical Components of PCR
The name ‘Polymerase Chain Reaction’ represents the nature of the process. ‘Polymerase’ because DNA Polymerase III is required for constructing new DNA strands, just like in a living cell. ‘Chain Reaction’ describes repeating cycles of replication which target a specific segment of a chromosome and use a “copy the copies” progression each cycle that doubles the amount of DNA copies of a specific segment of DNA present each cycle. In just 20 cycles of the chain reaction, over one million (220) copies of that specific segment of DNA can be produced. This is enough DNA to see with your naked eye. The goal of PCR is to make millions of copies of a specific segment of DNA that all originate from a single DNA sample.
The five chemical components that must be added to a test tube for the PCR reaction to work, include a DNA template, DNA polymerase III enzyme, single stranded DNA primers, nucleotides, and reaction buffer.
- The DNA template is a sample of DNA that contains the target sequence of DNA for copying.
- DNA pol III. There are two requirements for a suitable DNA polymerase enzyme for PCR. First, the enzyme must have a good activity rate around 75°C. Second, the enzyme should be able to withstand temperatures of 95-100°C without denaturing and losing activity.
- Two primers. Primers are short oligonucleotides of DNA, usually around 20 base pairs in length. Because the purpose of PCR is to amplify a specific section of DNA in the genome, such as a known gene, then primers of specific sequences must be used. The geneticist planning the PCR reaction will design a forward primer to bind to one strand and a reverse primer that complements and binds to the other strand. The primer design process to select forward and reverse primers is requiring appropriate genetics thinking and is describe later in this reading.
- The four different deoxyribonucleotide triphosphates (dNTPs). Adenine (A), guanine (G), cytosine (C), and thymine (T) are needed to provide the building blocks for DNA replication. DNA polymerase will add each complementary base to the new growing DNA strand according to the original strand’s sequence following normal A-T and C-G pairings.
- Finally, a reaction buffer. This creates a stable pH and provides the Mg2+ cofactor needed for DNA pol III activity.
Three Temperature Cycles
A key insight to the success of PCR as an in vitro DNA replication process which generates millions of specific sequence copies was a three-temperature cycle which accomplish three parts of DNA replication: denaturation of the double stranded template, annealing of the primers to the single strands and extension of new strand synthesis by DNA pol III.
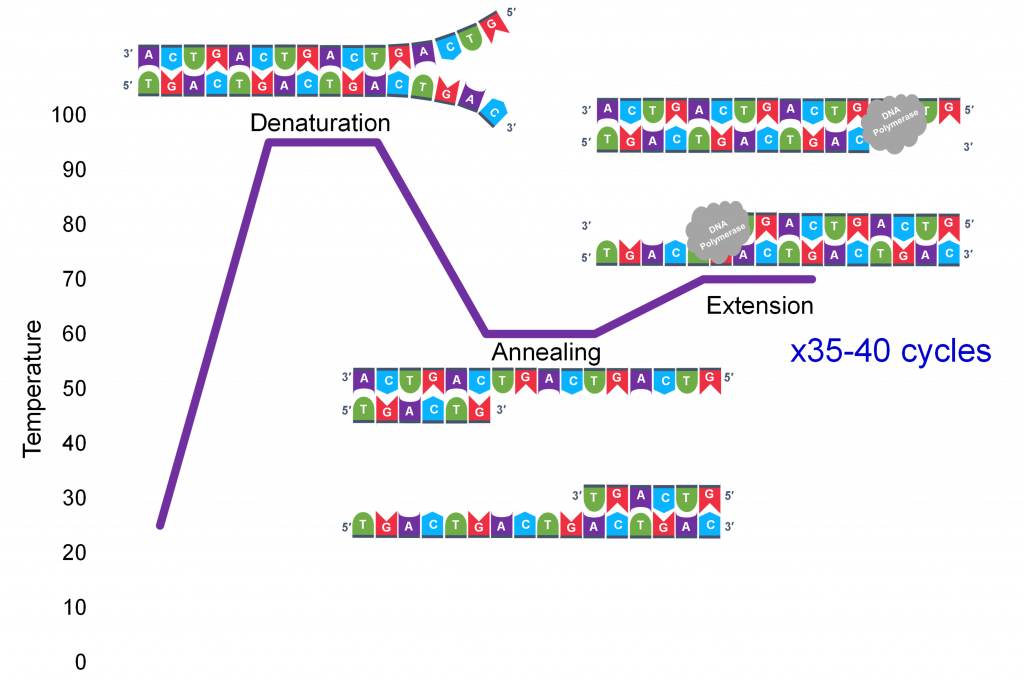
In general, a single PCR run will undergo 25-35 cycles. The first step for a single cycle is the denaturation step, in which the double-stranded DNA template molecule (Figure 2) is made single-stranded (Figure 3). The temperature for this step is typically in the range of 95-100°C, near boiling. The high heat breaks the hydrogen bonds between the strands but does not break the sugar-phosphate bonds that hold the nucleotides of a single strand together (Figure 3).

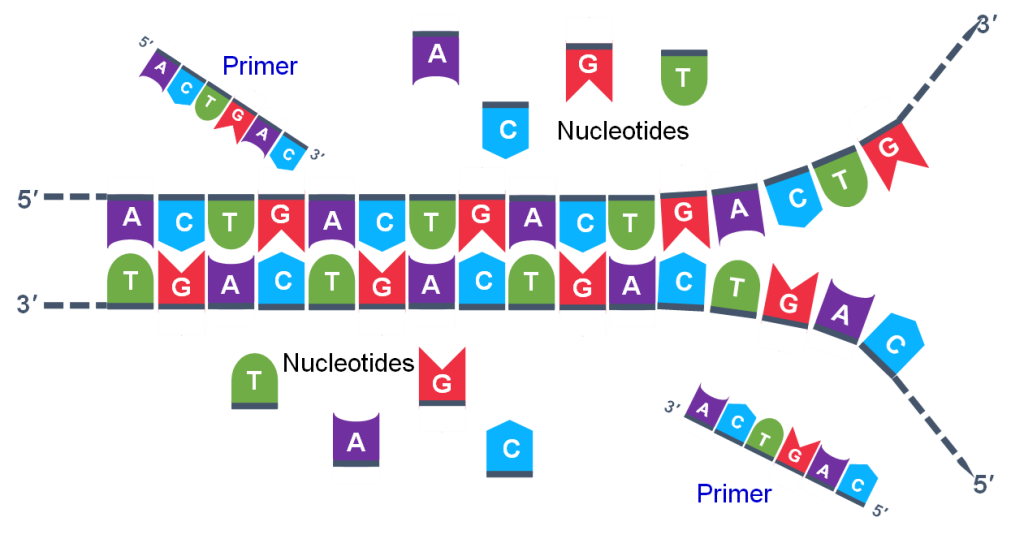
Thousands of copies of the single stranded primers and the individual nucleotides were added to the test tube prior to beginning the cycles. Both the primers and nucleotides will become part of the new DNA strands. The second step in the PCR reaction is to cool the temperature in the test tube to 45-55°C. This is the primer annealing step in which the primers bind to complementary sequences in the single-stranded DNA template. The two primers are called the forward and the reverse primer and are designed because their sequences will target the desired segment of the DNA template for replication (Figure 4).
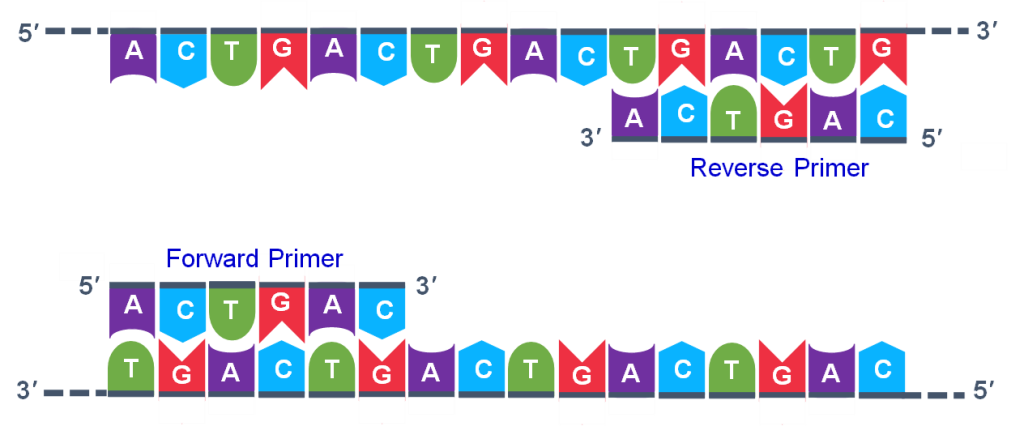
The geneticist planning the PCR analysis must “design” the forward and reverse primers and then buy them from a vendor who can synthesize single stranded DNA that has a specific sequence and length. The two most important criteria for primer design are the following.
- One primer must have a sequence that complements one of the template strands and the other primer must be complementary to the other strand. BOTH strands need to be primed for the replication process.
- The primers must bind so that their 3′ ends are ‘pointing’ in the direction of the other primer. This ensures that the sequence between the primers is replicated in the PCR cycles.
Extension: The final PCR step is when the DNA polymerase enzyme reads the template and connects new nucleotides to the primer’s 3’ end, extending a new complementary strand of DNA (Figure 5). Completion of the final step and the first cycle of PCR, will make two double stranded DNA copies from the original template DNA, doubling of the amount of DNA present. The test tube is heated to around 75°C, optimizing DNA pol. III activity and the newly synthesizing DNA strand is extended as the template strand is read by DNA pol. III. The Extension step will run for a few minutes and this step completes one PCR cycle.
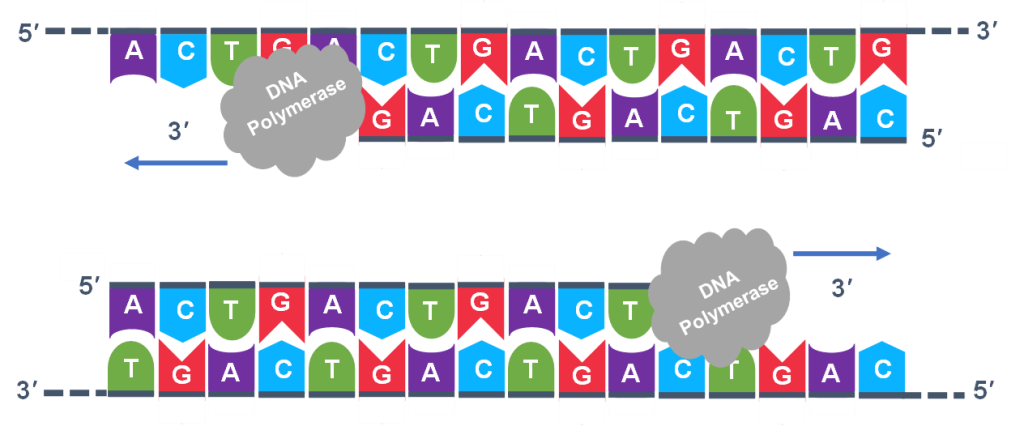
For cycle 2, the denaturation, annealing, and extension steps are repeated (Figure 6 a, b, c). This time, though there will be twice as many DNA template molecules compared to what there was at the beginning of cycle 1. Copies are being made by reading the original template and copies are made by reading the copies made in the previous cycle. Therefore, if everything is working correctly, the DNA replication in the test tube is a chain reaction where at the end of a cycle, there is double the amount of that DNA sequence as what was found at the beginning of the cycle.
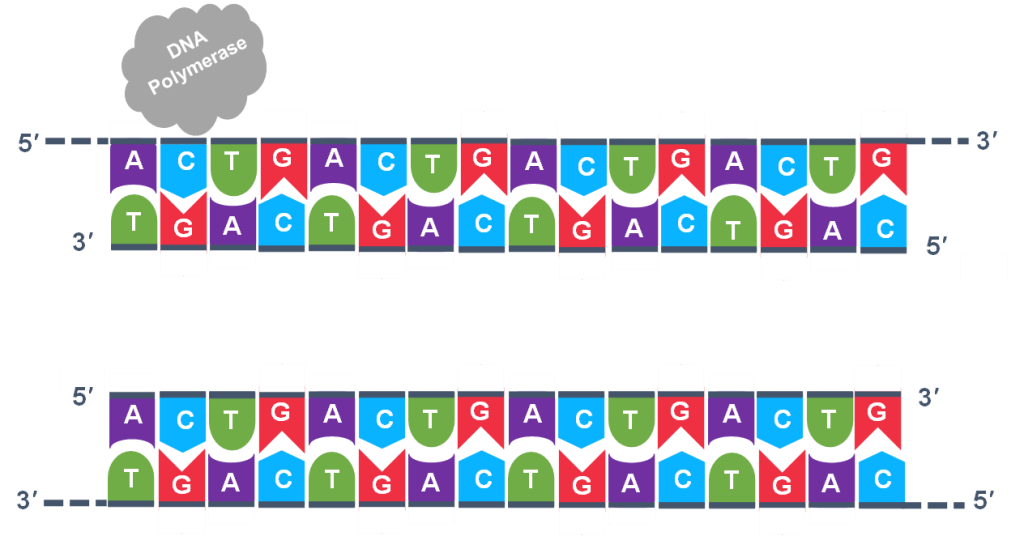
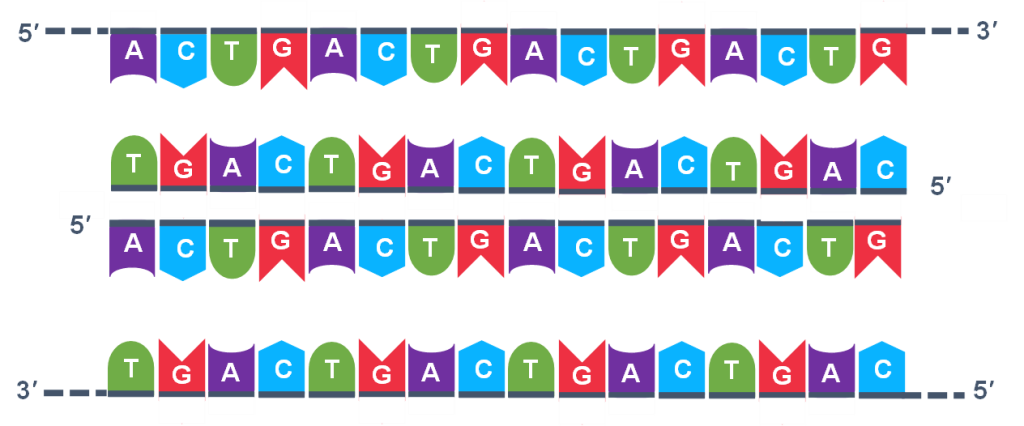
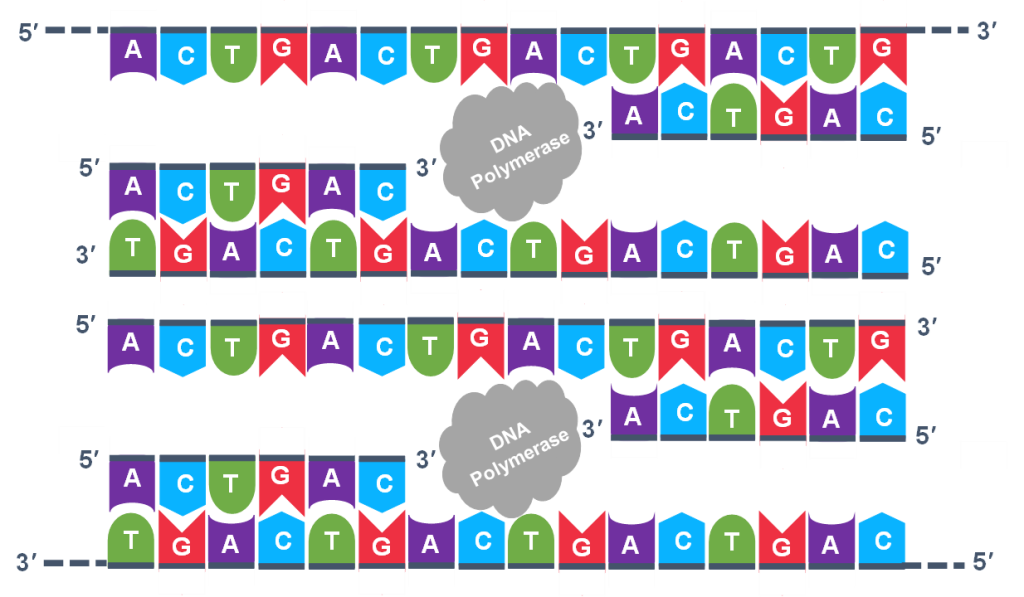
Because thousands of copies of the forward and reverse primer are added at the start of PCR, all the single strand templates, both the original, the copies in cycle 3 and beyond, and the copies of the copies made from previous cycles will be primed for the extension step of the cycles.
Thermal cycler
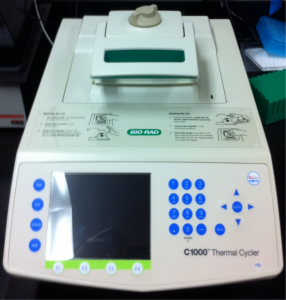
The instrument which heats and cools the DNA samples is called a thermal cycler (Figure 7). Each small tube or sample well in a plate contains all the chemical components needed for a PCR reaction. Adding a specific sample to the reaction mix provides the template DNA. A thermal cycler can be programmed for specific temperatures and the amount of time spent at each temperature. The engineered design of thermal cyclers to maximize the accurate replication of the targeted DNA in a small sample volume with the minimum amount of time can be critical in many applications of PCR.
Taq DNA polymerase
The Taq version of DNA pol III comes from Thermus aquaticus, (Taq) a thermophilic eubacterium found in hot springs (Chien et al. 1976) and does not easily denature in the hot temperatures required in PCR; plus, it has a good efficiency, able to add 60 base pairs/sec at 70°C. Like all other DNA polymerases, Taq DNA pol III cannot begin DNA replication without the addition of a starting primer.
Visualizing the Results with Electrophoresis
Once a PCR reaction has been completed, we need to be able to see the results. To do this, a sample of the PCR mixture is loaded into an agarose gel for electrophoresis. The agarose gel contains a matrix of pores which enables it to separate DNA fragments based on their sizes. For details about setting up and running an electrophoresis gel, see Electrophoresis: How Scientist observe fragments of DNA
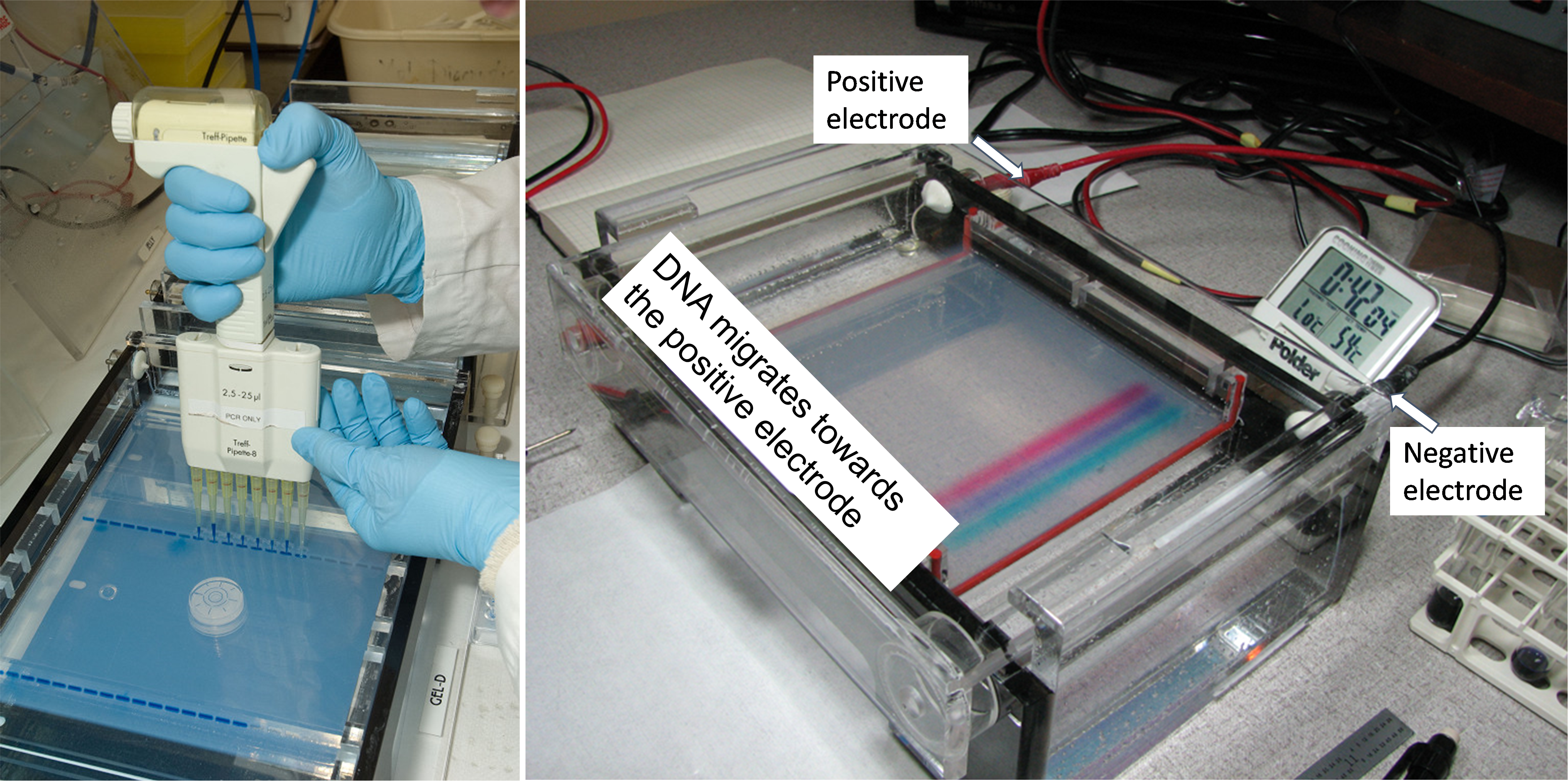
Figure 8 shows a picture of a gel electrophoresis gel that is running. The box on the right contains DNA loaded in the agarose gel. The gel placed in an aqueous solution of electrolytes. Depending on the type of dye used, color bands are a dye that was added to the PCR sample before it was loaded into the sample well. This allows for the tracking of the DNA’s progression through the gel. Hooked up to this gel unit is an electrical power source which provides the force to move the DNA through the gel. Since DNA molecules are negatively charged, they will migrate towards the red, positive electrode. Shorter DNA fragments move faster than longer fragments through the pores in the gel.
After the gel is run, the DNA is stained with a chemical that binds specifically to DNA molecules and then will either reflect a specific color of visible light or fluoresce a specific color when viewed with ultraviolet light. A single ‘band’ contains 1000s of individual DNA fragments, all of the same length. Figure 10 illustrates the visual information that can be obtained from an electrophoresis gel after it has run. The electric current uniformly moves all the DNA fragments through a gel in the same direction. The sample wells at the top of the gel image thus establish lanes for the DNA samples to move.
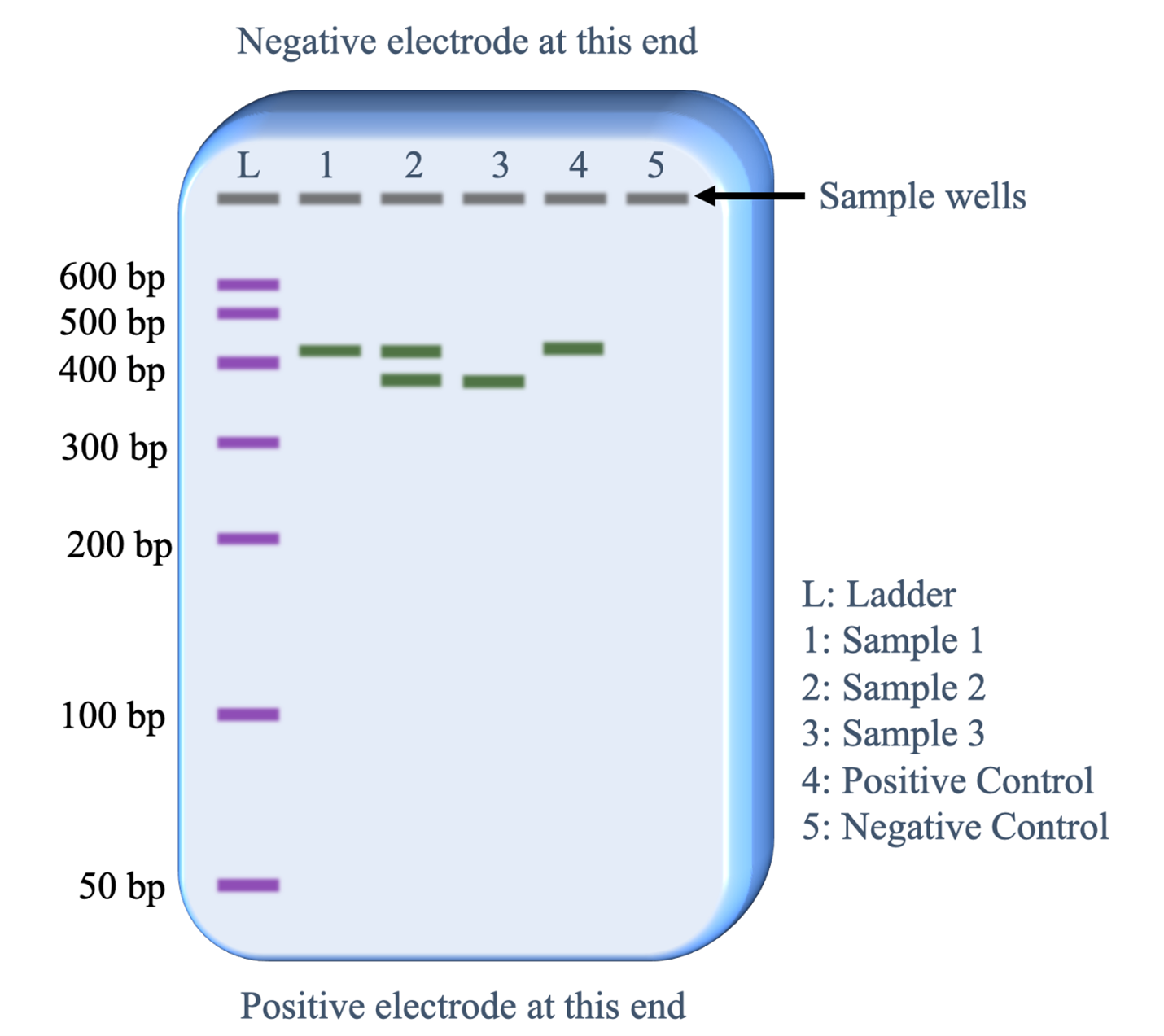
Below is a description of what information is revealed from each lane.
- Lane L: This was loaded with the DNA size ladder that contains copies of seven different lengths of DNA fragments. Commercial vendors of the DNA size ladder provide information on the lengths of each fragment in base pairs (bp). Running this lane provides an estimate of the DNA fragment lengths in the sample lanes (1-5).
- Lane 1: The PCR sample loaded in this lane has copies of a single length of DNA. The length is slightly more than 400 base pairs.
- Lane 2: The PCR sample loaded in this lane has copies of two lengths of DNA. One fragment is the same length as the fragments in lane 1 and the second fragment is slightly less than 400 bp.
- Lane 3: The PCR sample loaded in this lane has copies of one fragment that is the same length as the shorter fragments in lane 2.
- Lane 4: The positive control worked as predicted. The sample was set up with all the same reagents as the other PCR samples plus a DNA template that was known to contain the sequence targeted by the PCR primers that would generate a 410 base pair fragment.
- Lane 5: The negative control worked as predicted. The sample was set up with all the same reagents as the other PCR samples except no template DNA was added. Therefore, we would not expect the PCR reaction to work and the absence of a band of DNA fragments is as expected.
Because the positive and negative controls worked as expected, the biologist can be confident that the bands of DNA observed in lanes 1,2 and 3 reveal genetic information about the individual providing that DNA sample.
Advantages of PCR
PCR quickly became the method of choice for many types of DNA analysis because of several advantages over other DNA detection methods. The first; it is a simple procedure to set up and run. Fewer steps save time in getting a DNA analysis result. The second is the sensitivity. A very small amount of template DNA in the sample can be detected. Even just a few skin cells from one human hair contain enough DNA, making PCR useful in forensics. The third is that it can be designed to accurately differentiate genetic samples that are different by as little as single nucleotide in the targeted sequence. Finally, in application where large numbers of samples need to be subjected to the same PCR analysis, automation and robotic assistance allow the processing of many samples in a very short time. For example, an automated PCR would be critical for testing large numbers of people in hours during a pandemic.
PCR Adapted from Genetics, Agriculture, and Biotechnology Copyright © 2021 by Walter Suza; Donald Lee; Marjorie Hanneman; and Deana M. Namuth is licensed under a Creative Commons Attribution-NonCommercial-ShareAlike 4.0 International License, except where otherwise noted.